Prof. Dr. Philip Warren Anderson > Research Profile
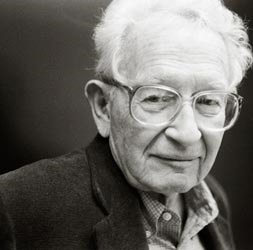
by Roberto Lalli
Philip Warren Anderson
Nobel Prize in Physics 1977 together with Sir Nevill F. Mott und John H. van Vleck "for their fundamental theoretical investigations of the electronic structure of magnetic and disordered systems".
Learning Physics During World War II
Philip Warren Anderson was born in Indianapolis on December 13, 1923. He belonged to a family of midwestern academics: His father was a Professor of plant pathology at the University of Illinois at Urbana-Champaign, and his maternal grandfather was a professor of mathematics in Crawfordsville, Indiana. Growing up in such an intellectually stimulating environment had a strong impact on the future interests of the young Anderson. Interested in the natural sciences, Anderson pursued his secondary education at the highly selective University of Illinois High School (Uni High for short), where he immersed himself in mathematical studies with the encouragement of a first-rate mathematics teacher.
In 1940, Anderson enrolled at Harvard University to major in mathematics with the support of a newly established scholarship. Convinced by his parents’ friend Wheeler Loomis—the head of the physics department at the University of Illinois—and attracted by Wendell Furry’s lectures at Harvard, Anderson eventually decided to switch to applied physics when he was still a freshman. In the pre-war period, students interested in natural sciences were urged to focus on topics useful to war-related research activities. Although the United States would enter World War II only at the end of 1941, military preparation already began in 1940. Among these preparatory activities, one of the most pressing was the training of scientific manpower to cope with subjects that could be immediately employed during the war. Following this general trend, Anderson chose to study electronic physics and graduated in this subject in early 1943. From 1943 to October 1945, he actively participated in the war related research endeavours of the U.S.A. as a member of the staff of the Naval Research Laboratory in Washington, D.C., where he worked on the construction of antennas.
PhD at Harvard on chemical physics
Soon after the end of the war, Anderson decided to pursue graduate studies in theoretical physics. The chairman of the Harvard Physics Department—the American theoretical physicist John H. van Vleck—had met Anderson during the war and made the necessary efforts to guarantee his return to Harvard as a PhD student in October 1945. During his PhD, Anderson learned quantum mechanics and nuclear physics for the first time, thanks of the teaching of Furry and, especially, Julian Schwinger—who was working on the development of the new methods for the renormalization of quantum electrodynamics that would gain him the 1965 Nobel Prize in Physics, along with S.-I. Tomonaga and R. Feynmam. During his PhD studies, Anderson had only one course on solid-state physics, which was taught by the elderly French physicist Léon Brillouin—famous for having introduced the Brillouin zones in 1930.
Still, Anderson became very interested in topics that would later be considered as part of the not-yet-defined field of condensed matter physics. He decided to write a dissertation on chemical physics, especially because he did not want to follow the general trend of the rapidly increasing interest in nuclear and particle physics and was not interested in working on highly formalistic research to cope with quantum electrodynamics problems. Consequently, Anderson chose van Vleck as supervisor instead of the more fashionable Schwinger.
Van Vleck assigned him a problem on the theoretical explanation of the detailed ammonia spectrum obtained with microwave spectroscopy, which was a field greatly improved by research activities done during wartime. In 1949, Anderson was able to solve the problem by also employing theoretical tools that he had learned from Schwinger. The results of his PhD research were published in a series of papers, the most important of which was “Pressure Broadening in the Microwave and Infra-Red Regions,” which became very influential for explaining of the breadth of spectral lines in the infra-red region, using methods for solving quantum scattering problems for molecule collisions under a series of assumptions on the relative motion of molecules as well as on the collision between them.
Research on magnetism at Bell Labs
After Anderson completed his dissertation, he began looking for a job. He had a strong preference for research laboratories of industrial firms. In particular, he wished to join the AT&T Bell Telephone Laboratory (Bell Labs for short), for during wartime he had been impressed by the quality of its researchers and of the devices it manufactured. Moreover, Anderson knew that one of the greatest achievements in communication technology—the discovery of the transistor—had been realized by Bell Labs physicists just a couple of years earlier. Recognizing the value of Anderson’s dissertation, Van Vleck heartily recommended him to the Bell Labs management. One of the inventors of the transistor—W. Shockley, who was also the leader of a Bell Labs sub-department devoted to the study of solid-state physics—soon hired Anderson in order to strengthen the theoretical section of his already successful group.
When Anderson joined, Bell Labs was at the forefront of theoretical research on solid-sate physics: Several first-rate experts of the field were permanently working there and the laboratory could benefit from many academics who either worked there for shorter terms as consultants or gave lectures for its staff. Anderson fruitfully used the knowledge that Bell Labs had been accumulating in this subject. His first months were spent in learning solid-state physics from Shockley himself and other members of Bell Labs theoretical staff.
Shockley soon asked him to work on calculations concerning the properties of ferroelectrics, which Anderson did in the first weeks after he was hired. However, after the first experiments on anti-ferromagnetism with neutron diffraction, Anderson got interested in the theoretical properties of anti-ferromagnetism. Refining a 1934 proposal by the Dutch theoretical physicist Hans Kramers on the long-range exchange interactions and working with Charles Kittel, Anderson put forward a theory of anti-ferromagnetism in the paper “Antiferromagnetism. Theory of Superexchange Interaction,” published in 1949. The superexchange is the strong magnetic coupling of two next-to-nearest positive ions through the doubly negative ions to which they have donated one electron each. Anderson’s work became rapidly influential and he was invited to give a talk at the meeting of the American Physical Society in 1950. In the Bell Labs environment, presenting an invited paper at the APS meeting was a valuable achievement. This success helped Anderson to preserve his position within the Bell Labs, despite the worsening of his professional relationship with Shockley, who demanded that Anderson immerse full time in the task Shockley had assigned him.
Anderson continued to work on the theory of anti-ferromagnetism in the following years and in 1952 he put forward a quantum theory of the antiferromagnetic ground-state, which was a posteriori seen as an application of the mechanism of spontaneous symmetry breaking. At the time, however, this feature was not recognized, even by the author himself. As Anderson recalled, he considered this work as a straightforward extension of some recent theoretical advances done in the field of ferromagnetism. At the same time, solid-state physics was gaining momentum within the larger physics community, and Anderson was being perceived as one of the young leaders of this field in the United States.
Following these important results, Anderson became the first physicist to win a Fulbright fellowship to go to Japan. He spent a semester at the University of Tokyo working with the Japanese physicist Ryogo Kubo, who would soon make fundamental contributions to the theory of non-equilibrium statistical mechanics. When he came back to Bell Labs, Anderson continued to work on the magnetic properties of solids and produced one of the two research achievements that would gain him the Nobel Prize some twenty years later. Anderson began working as a theoretical consultant with a group of Bell Labs experimenters led by G. Feher who were making observations on paramagnetic resonances as a function of impurity concentration.
A problem Anderson had to face was whether there was a sort of impurity band or whether the wave functions of the impurities were separated from the bands of the original material. After two years of intellectual struggle, Anderson realized that the experimental data showed a sort of localization, which became a central feature of his theoretical model for disordered systems. Put forward in the 1958 paper “Absence of Diffusion in Certain Random Lattices,” the strong localization model—as the Anderson model was also called—described processes such as spin diffusion—i.e., the transfer of nuclear spin polarization—and conduction as quantum jumps between localized states. Anderson derived the conditions necessary for a disordered medium to allow for such diffusion. To explain the absence of spin diffusion or conduction under certain conditions, Anderson showed that, in his quantum mechanical model, if the degree of randomness of impurities is sufficiently large, the electrons of semi-conductors remain localized. As Anderson stated in his Nobel Lecture, the fundamental conceptual step in his breakthrough was to deal with distributions rather than with averages. This implied that random systems were to be treated in a fundamentally different manner than normal lattices.
For some years, the reaction to Anderson’s theory was rather unsympathetic. It introduced new concepts that were hard to digest, and its implications were not perfectly clear even to the author himself. It was thanks to the work on disordered systems of the British solid-state physicist Nevill Mott and a series of experimental verifications of these theoretical developments that Anderson’s 1958 paper came to be regarded as a fundamental step in the understanding that in non-crystalline systems the lowest states in the conduction bands are localized.
Superconductivity, spontaneous symmetry breaking and the Higgs mechanism
After J. Bardeen, L. Cooper and J. Schrieffer had put forward the first successful microscopic theory of superconductivity in 1957, Anderson got interested and was immediately persuaded that it was the right theory of superconductivity. On the experimental side, the BCS theory—as the Bardeen-Cooper-Schrieffer theory was soon called—was very successful. On the theoretical side, however, some physicists voiced serious doubts about its validity. The most important of these criticisms focused on the lack of manifest electromagnetic gauge invariance of the BCS superconducting ground state. Bardeen, Cooper, and Schrieffer had, indeed, deduced the Meissner effect—the sudden expulsion of the magnetic field from the superconducting material when it is cooled down below its critical temperature—with a specific choice of gauge, divA=0. Bardeen was, however, convinced that the BCS theory became gauge invariant if one also took into account the collective longitudinal modes. Anderson made important contributions to this debate by clarifying the connection between the BCS theory and what is now called the spontaneous symmetry breakdown of gauge symmetry.
Convinced of the validity of the theory because of its good empirical evidence, Anderson began researching a formalism that would make the BCS theory manifestly gauge invariant. Anderson realized that it was necessary to add a space-time dependent quantum field, which described the longitudinal collective excitations. One then could write a Hamiltonian that was locally gauge invariant if the added quantum field changed in dependence on the gauge transformation of the wave function. Elaborating on these intuitions, Anderson put forward various ways in which the BCS theory could overcome the gauge invariance problem in three papers published in 1958. In the second one, “Random-phase Approximation in the Theory of Superconductivity,” Anderson developed the spinor representation of the BCS theory, which allowed an essentially complete elaboration of the mechanism later described as spontaneous symmetry breaking. Some Soviet Physicists, including L. Gorkov and N. Bogoliubov, also independently re-derived the BCS theory in a formalism that made it manifestly gauge invariant. All these theoretical advances solved most of the problems that had been raised against the BCS theory. Also because of these theoretical clarifications, the BCS theory shortly became the standard theory of superconductivity.
As an important by-product, the mechanism created in order to overcome the gauge problem in the BCS theory would become of fundamental importance in the field of elementary particle physics starting from some articles by Y. Nambu and G. Jona-Lasinio published in 1960 and 1961. In these papers, the authors explicitly used the analogy with superconductivity to propose that the mass of nucleons arose from the spontaneously broken chiral symmetry of more fundamental particles. Anderson also played an important role in the transfer of the spontaneous symmetry breaking mechanism from the field of solid-state physics to that of particle physics in what is now called the Higgs mechanism, which is sometime referred to also as the Anderson-Higgs mechanism (along with many other different names).
In 1961, J. Goldstone proved a theorem according to which the spontaneous breakdown of a generic continuous symmetry of a Lorentz-invariant field theory necessarily resulted in the formation of massless bosons (later called Goldstone or Nambu-Goldstone bosons). Theoretical particle physicists were worried by the implications of this theorem because no massless bosons except for photons had ever been observed. The theorem, as a consequence, seemed to be a serious limit to the possibility that gauge quantum field theories could represent a reliable framework for the description of particle interactions. The problem was that the non-Abelian Yang-Mills gauge field theories implied the existence of a massless boson. The only way to avoid this empirically problematic feature was to suppose that the original global symmetry of the Yang-Mills Lagrangian was spontaneously broken and, consequently, that the vector bosons were made massive by this symmetry breakdown. The Goldstone theorem, which was rigorously proved by Goldstone, Salam and Weinberg in 1962, seemed to deny this possibility.
Anderson, however, recognized that his 1958 paper on superconductivity gauge invariance already contained the resolution of this problem: In the BCS theory there was no massless Goldstone bosons because the gauge-symmetric bosons combined with them to create massive vector bosons—the plasmons representing the longitudinal collective excitation. In 1962, Anderson explicitly tried to translate these ideas into the language of particle physics by setting up a mechanism that showed that Goldstone bosons could tangle with the Yang-Mills gauge bosons and, then, form massive vector bosons. In this way, he argued that the Goldstone theorem was not such a fundamental difficulty to the acceptance of the Yang-Mills gauge theories as a theoretical framework for the strong nuclear interactions. This mechanism, which Anderson summarized in the paper “Plasmons, Gauge Invariance, and Mass,” was shortly elaborated by P. Higgs and, independently, re-discovered by other theoretical physicists in an explicit relativistic model. In 1967, this mechanism became an essential element of the SU(2)xU(1) gauge theory of electro-weak interactions, which, after further theoretical developments and relevant empirical confirmation, became one of the two pillars of the Standard Model of particle physics in the 1970s. After the massive Higgs boson foreseen by the theory was eventually discovered at the CERN’s Large Hadron Collider in 2012, two of the various physicists who had put forward the mechanism in 1964—P. Higgs and F. Englert—were awarded the 2013 Nobel Prize in Physics. While the Higgs mechanism became a fundamental piece of the Standard Model of particle physics in the 1970s, Anderson was embittered by the fact that, in his view, his contributions had not received the credit they deserved within the particle physics community, although Higgs continued to acknowledge Anderson’s contribution.
Anderson’s work on the problem of the gauge invariance of the BCS theory was not his only contribution to the understanding of superconductivity and other collective phenomena. Anderson continued to be interested in the formal structure of the BCS theory as well as in its possible implications and extension. The most important of these achievements was perhaps the work Anderson began in 1962 with the French physicist Pierre Morel on realistic calculations of critical temperatures starting from first principles of the BCS theory.
This work was later extended after the discovery of the Josephson effect—the quantum tunnelling of Cooper pairs through an insulating layer. The young British theoretical physicist Brian D. Josephson—who was fascinated by Anderson’s lectures on superconductivity at Cambridge—predicted the supercurrent tunnelling effect in 1962. The prediction was very controversial, to the point that Bardeen strongly argued against its existence in public debates. Anderson, however, rapidly became one of its advocates and, along with the British experimental physicist John Rowell, published the first paper that discussed the experimental testability of the effect and claimed for its discovery. The experimental work on the Josephson effect was useful to develop accurate spectroscopic methods for the study of the structure of the energy band of superconductors. The experiments Rowell carried on at Bell Labs allowed Anderson to make actual correlations between the equations recently developed for the study of real materials and the empirical tunnelling curves and phonon spectra of lead. From this correlation, and thanks to an informal collaboration with a similar project carried out by Schrieffer and his group, Anderson and his co-workers developed a quantitative theory of critical temperatures. The work done by Anderson and his collaborators, along with the simultaneous one carried on by Schrieffer’s group, was the starting point of the “quantitative period of superconductivity theory,” in which physicists began employing the developed tools to accurately calculate the relationship between critical temperature, resistivity, phonon spectrum and superconducting curves.
Anderson’s model of local magnetic moments
In the same period in which Anderson was immersing himself in the theory of superconductivity, and partially related to such efforts, he also made the second breakthrough in the field of magnetism that was quoted in the motivations of his Nobel Award in 1977: the Anderson model of local magnetic moments. The problem to theoretically describe the effects of impurities in solid-state physics greatly fascinated Anderson who became more and more involved. One of the most pressing questions concerned the way in which some materials, which in pure form were not magnetic, under certain circumstances show the presence of local magnetic moments. Anderson developed a simple quantum mechanical model that could explain several of the empirical features concerning the local magnetic moments of metals in dependence of the concentration of impurities.
This model was developed in 1961 as an extension of the theory of superexchange that Anderson had already applied successfully to insulators in 1959, by including the Coulomb correlation of the electrons in the inner shell states in the mathematical treatment. The schematic model Anderson put forward in 1961 allowed qualitative predictions about the appearance of the effect in solutions of specific metals. Since Anderson’s model used ideas that have been already part of the knowledge of the growing solid-state community, the theory was rapidly accepted and became a valuable tool to cope with real materials—a completely different reception with respect to the one that followed Anderson’s theory of localization about disordered systems. The two insights, however, presented important similarities in that they focused on the concept of local effects with respect to the general description of solid states based on the theory of bands.
All the insights previously summarized made Anderson a leading exponent of the solid-state community. A similarly important figure was the older Nevill F. Mott, who had been appointed Cavendish Professor at Cambridge in 1954 and was trying to redirect the Cavendish Laboratory from nuclear physics to solid-state physics in the early 1960s. Mott managed to have Anderson as visiting scholar at Cambridge University in the academic year 1961-1962. This initial collaboration was very fruitful and was re-proposed in 1967 to become a permanent visiting Professorship until 1975. This state of affairs produced an intense collaboration between Anderson and the Cavendish Laboratory. By the 1970s, Anderson’s various achievements obtained in the field of theoretical solid-state physics began to be recognized by the entire physics community. Thanks to Mott’s work, Anderson model of localization came to be regarded as an important step in the understanding of disordered systems. In the 1970s, various achievements obtained in the field of theoretical solid state physics were awarded with Nobel Prizes in Physics: L. Néel was awarded in 1970 for his work on ferromagnetism and antiferromagnetism; Barden, Cooper, and Schrieffer received the award in 1972 for the discovery of the BCS theory of superconductivity; the following year, Josephson was awarded for the theoretical prediction of the Josephson effect. In 1977, the various contributions Anderson made to the growth of this field, along with those made by Mott and Anderson’s PhD supervisor van Vleck, were also awarded with a Nobel Prize in Physics. The unusually generic motivation for this award referred to “their fundamental theoretical investigations of the electronic structure of magnetic and disordered systems.”
Emergence vs. Reductionism in Theoretical Physics
Anderson became a leading figure within the solid-state physics community not only because of his relevant scientific achievements, but also because he articulated and defended an anti-reductionist philosophical position in a debate that involved particle physicists and solid-state physicists about what had to be regarded as fundamental research in science. In 1971, Anderson published the extremely influential paper “More is Different,” in which he argued against the strong reductionist position, which regarded the search for the ultimate constituents of nature—and thus elementary particle physics—as the most relevant among scientific enterprises. To put things simply, the reductionist position maintained that the laws of complex systems were to be considered as epiphenomena due to the interaction of many such elementary entities. Although Anderson did not refer to the concept of emergence in his 1971 paper (because he did not know it yet), his paper rapidly became a manifesto of emergence in science. This anti-reductionist perspective considered the properties of each layer of complexity to be largely independent from the laws governing the lower levels. To make his case, Anderson argued that superconductivity—which in the BCS theoretical framework was understood as a consequence of the spontaneous breakdown of gauge symmetry—was a striking example of emergent properties that cannot be anticipated by the study of the more simple entities constituting the system.
The two positions had many nuances, which were discussed in a harsh debate by many scientists including a number of theoretical physicists. Unsurprisingly, the advocates of a more reductionist view were to be found within the ranks of particle physicists, while solid-state physicists usually held an anti-reductionist perspective. This philosophical debate had some very pragmatic consequences, because it was explicitly connected to government science policies concerning the funding of specific research enterprises, especially in the United States. In fact, the debate dramatically evolved in relation to the project to build the Superconducting Super Collider (SSC), which was planned to become the most powerful particle accelerator in the world. Since 1982, when American experimental particle physicists proposed the enormously expensive project, Anderson began arguing vociferously against it on the grounds that the planned 4.4 billion dollars needed to build the SSC would be much better utilised in other field of physics, such as condensed matter physics, which would indubitably have a greater and more immediate impact on human life. In 1987, before the final approval of the project by the U.S. administration, Anderson testified before the U.S. Congress against the usefulness of the SSC. In spite of Anderson’s fierce disparagement, the U.S. Congress approved the construction of the SSC in 1987, only to eventually suspend it in 1992 because of the changed geopolitical climate, especially due to the end of the Cold War.
Later Work on Superconductivity
After Anderson received the 1977 Nobel Prize in Physics, he continued to make important contributions to the fields of magnetism, disordered systems, and superconductivity. He was also involved in the attempts to provide theoretical explanations for an important discovery made at IBM in 1986; namely, that some insulating materials become superconductors at unusual high temperatures. The surprising discovery was soon confirmed by another experimental group that observed the passage from a normal to the superconducting state at an even higher critical temperature. This rapid confirmation led the Royal Swedish Academy of Sciences to award the 1987 Nobel Prize in Physics to Georg Bednorz and K. Alex Müller for “for their important break-through in the discovery of superconductivity in ceramic materials.” The same year, Anderson put forward a theoretical model for the description of high-temperature superconductivity in copper oxides (now called cuprates), and from that year he was involved almost exclusively in that field. The theory—called resonating valence bond theory—predicts that, in cuprates, pairs of neighbouring copper atoms become linked by means of chemical valence bonds, sharing electrons with opposite spins. While the electron pairs usually remained localized, the doping of the material might free the electrons that then act as Cooper pairs below a certain temperature. The theory had a good reception, but the theoretical status of high-temperature superconductivity has remained highly controversial up to this day. Anderson’s theory is still one of the two theoretical frameworks on which physicists most rely, the other one being the theory of spin fluctuation that P. Monthoux, A. Balatsky, and D. Pines proposed as an explanation of high temperature superconductivity in 1991.
His long-lasting commitments to the properties of many-body systems led Anderson to become involved as a Vice-Chairman and external professor in the Santa Fe Institute—a theoretical research institute devoted to the theoretical study of complex adaptive systems founded in 1984 by several scientists including the American theoretical physicists M. Gell-Mann and D. Pines. Anderson’s view of complexity is strongly related to his anti-reductionist philosophical position, and he is still dedicating his intellectual energy to promote those fields that in the near future will probably cross the boundaries between different disciplines.
Bibliography
Anderson, P. W. (1977) Local Moments and Localized States. In Nobel Lectures, Physics 1971-1980, Editor Stig Lundqvist, World Scientific Publishing Co., Singapore, 1992, pp. 376-398.
Anderson, P. W. (1994) A Career in Theoretical Physics. World Scientific, Singapore.
Anderson, P. W. (2005) Philip W. Anderson - Biographical. Nobelprize.org. Nobel Media AB 2014. Retrieved 14 December 2014. http://www.nobelprize.org/nobel_prizes/physics/laureates/1977/anderson-bio.html
Anderson, P. W. (2011) More and Different. World Scientific, Singapore.
Brown, L. M., & Cao, T. Y. (1991). Spontaneous breakdown of symmetry: its rediscovery and integration into quantum field theory. Historical studies in the physical and biological sciences, 21, pp. 211-235.
Castellani, E. (2002) Reductionism, Emergence, and Effective Field Theories. Studies in History and Philosophy of Modern Physics, 33, pp. 251-267.
Cat, J. (1998) The Physicists’ Debates on Unification in Physics at the End of the 20th Century. Historical Studies in the Physical and Biological Sciences, 28, pp. 253-300.
Cooper L. N., & Feldman, D. (eds.) (2011) BCS: 50 years. World Scientific, Singapore.
Hoddeson, L., Braun, E., Teichmann, J., & Weart, S. (eds.) (1991) Out of the Crystal Maze: Chapters from the History of Solid-State Physics. Oxford University Press, New York.
Hoddeson, L., Brown, L. M., Riordan, M. & Dresden, M. (1995) The Rise of the Standard Model: Particle Physics in the 1960s and the 1970s. Cambridge University Press, Cambridge.
Karaca, K. (2013) The construction of the Higgs mechanism and the emergence of the electroweak theory. Studies in History and Philosophy of Modern Physics, 44, pp. 1-16.
Interview of Philip Anderson with Alexei Kojevnikov. Niels Bohr Library & Archives, American Institute of Physics, College Park, MD USA. Retrieved 14 December 2014.
http://www.aip.org/history/ohilist/23362_1.html
Legget, A. J. (1995) Superfluids and Superconductors. In Brown, L., Pippard, B., & Pais, A. (Eds.). Twentieth century physics (Vol. 2). AIP, New York, pp. 913-966.
Ong, N. P., & Bhatt, R. N., (2001) More is different: Fifty Year of Condensed Matter Physics. Princeton University Press, Princeton.
Riordan, M. (2000) The Demise of the Superconducting Super Collider. Physics in Perspective, 2, pp. 411-425
Schweber, S. S. (1993) Physics, Community, and the Crisis in Physical Theory. Physics Today, 46, pp. 34-40.