Prof. Dr. Sir Aaron Klug > Research Profile
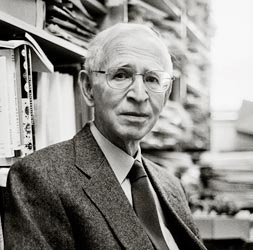
by Luisa Bonolis
Aaron Klug
Nobel Prize in Chemistry 1982
"for his development of crystallographic electron microscopy and his structural elucidation of biologically important nucleic acid-protein complexes".
X-ray crystallography and virus structures
Aaron Klug was born in 1926 in Zlvas, Lithuania, but he spent his early days in Durban, South Africa, where his family moved when he was two years old. While attending Durban High School he developed an interest in science, especially after reading Microbe Hunters, a popular book written by Paul de Kruif. As a way into microbiology he studied medicine for a year, but he was disappointed by the lack of fundamental science and moved first to chemistry and later to physics and mathematics. In 1945, he finally took a science degree with first-class honours at the University of Witwatersand in Johannesburg. By then he had decided that he wanted to do research in physics and moved to the University of Cape Town where he obtained the degree of M.Sc. in 1946 and where he remained two years as a junior lecturer. During this period he was particularly influenced by R. W. James, from whom Klug learned the techniques of X-ray crystallography, the method of recording on a photographic plate the patterns produced when a beam of X rays passes through a crystal. The phenomenon of X-ray diffraction hypothesised in 1912 by Max von Laue was immediately confirmed by experimental tests and soon Henry Bragg and his son Lawrence Bragg developed it into a technique for the analysis of crystals. James had brought to Cape Town the traditions of the Bragg school at Manchester and was an excellent teacher. Klug collaborated with him at X-ray diffraction studies of small organic compounds, a research during which he developed a method of using molecular structure factors for solving crystal structures and studied some quantum chemistry to calculate bond lengths. From this work he derived a strong interest in the structure of matter.
In 1949 Klug moved to England with a British 1851 Exhibition Scholarship and a research grant from Trinity College, Cambridge. He hoped to have a position at the Unit for Molecular Biology in the Cavendish Laboratory with Max Perutz and John Kendrew, both using X-ray crystallography in an effort to determine the structure of large protein molecules. At that time Francis Crick had just begun to work under Perutz and was supposed to study the structure of some small protein. Klug, however, was not so lucky. No openings were available, and Klug worked instead under D. Hartree - a former colleague of both Bragg and James at Manchester - on the molecular structure of steel, for which he received his doctorate in 1952. He learned a good deal during this time, particularly about computing and solid-state physics, and the idea of nucleation and growth during a phase transition had its echo when he came later to think about the assembly of Tobacco mosaic virus from its constituents.
His subsequent investigations at the colloid science department on the biophysical processes by which oxygen and carbon dioxide are exchanged in haemoglobin, further enhanced his interest in X-ray analysis of biological molecules. He decided that he really wanted to work on the X-ray analysis of biological molecules. Thanks to a Nuffield Fellowship he was able to move to Birkbeck College, London, under John Bernal, Perutz's former teacher at Cambridge. In 1934, Bernal had been the first to obtain clear images of X-ray diffraction patterns of the crystallized protein pepsin, becoming the founder of protein crystallography. In their initial work in the 1910s, the Braggs had applied the technique of X-ray analysis to relatively simple crystals, such as sodium chloride, that are composed of only a few types of atoms. Bernal, however, was interested in the far more complex structure of proteins, and he hoped that diffraction studies would eventually enable him to elucidate the function of a given protein. Stimulated by Bernal's visionary faith in the power of X-ray diffraction, in the late 1930s Perutz began investigating haemoglobin, the carrier of oxygen from the respiratory organs to the rest of the body. It was only the beginning of a long and ambitious research path. It took Perutz more than 20 years of hard work to solve the structure of haemoglobin.
First encounter with the Tobacco Mosaic Virus
When Klug arrived at Birkbeck College at the end of 1953, Rosalind Franklin had recently moved there from the King's College, where she had carried out X-ray analysis of DNA. Her excellent X-ray diffraction photographs had been instrumental in the final stages of Crick and Watson's race for the three-dimensional helical structure of the DNA molecule that had concluded in the spring of 1953. At Birkbeck College Franklin's research interest had turned to RNA, and specifically to the tobacco mosaic virus (TMV), a simple virus consisting only of a single type of protein molecule and of RNA, the carrier of the genetic information. X-ray studies of TMV had been begun by Bernal already in 1936. Viruses, which represent the border between living and inorganic matter, can be said to be genetic material without a cell of its own. Their structure provides clues to the more complicated organisation of the hereditary material in higher organisms. Plant viruses consist of a single strand of RNA enclosed in a protein coat. Such objects are highly symmetrical on account of economy of design. The coats are built up by the repetitive use of one kind of subunit. Two classes of object result: rod viruses such as tobacco mosaic virus, one of the simplest viruses known, and the so-called spherical viruses. At that time, when even solving the structure of a small protein was a formidable task, Franklin had embarked on X-ray diffraction analysis of complex virus structures, in particular she studied TMV, whose molecular structure had not yet been elucidated, as a vehicle for structured RNA. Fascinated by her project, Klug actively collaborated with her, playing the more theoretical role and in four years, together with Kenneth Holmes and John Finch, who had joined them as research students, Klug and Franklin were able to map out the general outline of the TMV structure.
Franklin set Klug the example of tackling large and difficult problems, introducing him to macromolecular assemblies, the biological organisation on a scale between the atomic and microscopic dimensions. After Franklin’s untimely death in 1958, Klug took over the leadership of the small research group working on plant-virus structure and became director of the Birkbeck Virus Structure Research group. Through Franklin, Klug had got to know Francis Crick with whom he published an important theoretical paper on helical diffraction theory. Klug's collaboration and friendship with Crick led in 1962 to the incorporation of his research group in the newly constituted Medical Research Council Laboratory of Molecular Biology in Cambridge, which, under the leadership of Perutz, was to house the original unit from the Cavendish Laboratory (Perutz, Kendrew, Crick, and, later, Brenner), enlarged by Frederick Sanger’s group from the Biochemistry Department and Hugh Huxley from University College London. Sanger had been recently awarded the Nobel Prize in Chemistry 1958 for his work on the structure of proteins, especially that of insulin. Almost half a century had elapsed before the technique of X-ray diffraction had developed to a point allowing the determination of the structure of the giant molecules that are the building blocks of life. The aim of their field of structural molecular biology was to describe the biological machinery in molecular, i.e., chemical, detail. The publication of the structure of haemoglobin at 6-angstrom resolution by Perutz and his collaborators along with Kendrew's findings on the myoglobin molecule at 2-angstrom resolution in Nature, in 1960, heralded a new era in biology, marked in 1962, when Max Perutz and John Kendrew were awarded the Nobel Prize for the first solution of the structure of proteins. In the same year, Francis Crick, James Watson, and Maurice Wilkins were likewise honoured for elucidating the structure of the double helix of DNA.
Virus particles: structure and assembly
Klug's first major contribution to molecular biology, together with Donald Caspar, a collaborator of Jim Watson in Yale, was to explain in 1962 how the protein shells of spherical viruses are constructed from large numbers of apparently identical subunits. In 1955, Klug and Finch had taken up the X-ray analysis of spherical viruses that had been investigated by Bernal's group just before and after World War II. With sufficiently high resolution X-ray and electron microscopy they found in more than one case that what appeared to be spherical viruses, actually displayed full icosahedral symmetry, arguably the highest symmetry shown in Nature. In 1956, Crick and Watson had proposed that small isometric viruses should form an isometric shell around the nucleic acid. The largest number of subunits that could be arranged symmetrically on the surface would be 60, with icosahedral symmetry, as was indeed indicated by X-ray patterns and electron microscope images. However, Caspar and Klug found that the number of subunits in most virus shells was considerably larger than 60. To resolve this dilemma, after reading about the large geodesic domes designed by the American engineer Buckminster Fuller, they imagined the virus shells spread out as flat hexagonal networks that could be cut and folded to form larger and larger icosahedra and proposed that the equivalent bonding required by exact symmetry could be relaxed to what they termed 'quasi-equivalence' and thus accommodate some multiples of 60 subunits. The concept of self-assembly underlying the principle of quasi-equivalence that had helped to interpret the structures of spherical viruses materialised in the surface patterns appearing in photographs of spherical viruses taken through an electron microscope.
Klug also studied the phenomenon of self-assembly both experimentally and theoretically in the case of the tobacco mosaic virus, the classical example of a rod-shape virus. After Franklin's death, Klug continued and extended its X-ray analysis with John Finch and Kenneth Holmes, who were then students, and who became colleagues. The determination of such a large structure by X-ray methods posed formidable technical and analytical problems. By the early 1960s, Klug came to realise that a way around this difficulty was to try to crystallise the isolated protein subunit of the virus, solve its structure by X-ray diffraction and then try to relate this to the virus structure solved to low resolution. The rod shape of TMV results from its basic design, namely a regular helical array of identical protein subunits, into which is embedded a single molecule of RNA wound as a helix. It is, of course, the RNA that carries the genetic information, i.e. the capacity to instruct the host cell to make many copies of the virus. The geometry of the protein arrangement forces the RNA backbone into a moderately extended single-strand configuration. Running up the central axis of the virus particle is a cylindrical hole with a 40-angstrom diameter, which they then thought to be a trivial consequence of the protein packing, but it turned out to figure prominently in the story of the assembly.
This general picture was already complete by 1958, when Rosalind Franklin died, and at first sight, it seemed easy to understand how a helical structure like that of TMV might be built out of identical subunits making identical contacts with its neighbours so that the bonding between them repeats over and over again, assembling themselves by repeated identical interactions. This would be like adding steps in a spiral staircase in a unique way, enclosing the RNA as a corkscrew-like thread as the rod extends. It was thus not surprising that tobacco mosaic virus could be reassembled from its isolated protein and nucleic acid components. By simple remixing, infectious virus particles were formed that were structurally indistinguishable from the original virus. Thus all the information necessary to assemble the particle must be contained in its components, that is the virus “self assembles”, a term that Klug himself introduced into the field.
The simple apparently satisfactory picture described above is actually wrong. When the RNA and the coat protein of the virus are taken apart, the assembly of the virus revealed a much more complex process than might have been expected from the simplicity of the helical design of the particle. In 1966, Klug and his collaborators discovered the existence of two juxtaposed layers, or rings, of 17 protein subunits each, that Klug named the two-layer disk. How could a closed-ring structure be related to the helix assembly? The structure had clearly a geometry related to that of the virus particle and the results coming from growing data at disposal made him wonder whether the disk aggregate might not be fulfilling some vital biological role.
Although the structure of the disk was solved in detail only in 1977, an earlier stage in the X-ray analysis gave the clue as to how it might interact with the RNA. The disk aggregate of protein actually had a number of significant properties that strengthened Klug's conviction that the disk form might play a significant role in the assembly of the virus. Assembly of any large aggregate of identical units, such as a crystal, can be considered from the physical point of view in two stages: first nucleation and then the subsequent growth. The problem was to understand how the mode of initiation, in which the free RNA interacts with individual proteins subunits, was getting started. The protein actually forms an obligatory intermediate, the cylindrical disk composed of two layers of protein units, in which the packing of the subunits differs somewhat from the arrangement in the complete virus containing both RNA and protein.
The disk is designed to permit the RNA to enter through the central hole, and the growth of the virus is initiated with the binding or attachment of the specific sequence of the viral RNA to the protein disk, leading to the recognition by the disk during the nucleation process.
These experiments showed that the formation of the protein disk is the key to the mechanism of the assembly of TMV. In representing an intermediate subassembly, the disk is both the nucleus - attracting an initiation sequence in the viral RNA - and the building unit for growth of the virus rod. It provided an extraordinary mechanism by means of which the difficult problem of simultaneously fulfilling the physical requirement for nucleating the growth of the helical particle and the biological requirement for specific recognition of the viral RNA.
Due to the crucial role that the protein disk plays in the assembly of the virus from its RNA and protein, the crystallographic analysis required the combined efforts of Klug and many co-workers, as well as new computer methods. This was the first very large structure ever to be tackled in detail by X-ray analysis and it took about a dozen years to carry through the analysis to high resolution. The formidable technical problems were overcome only after the development of more powerful X-ray tubes and of special apparatus (cameras, computer-linked densitometers) for data collection from a structure of this magnitude. In 1978, it was possible to construct an atomic model showing the detailed structure of the protein subunit and how it interacts with its neighbours and to elucidate the process of self-assembly of such partial structures to give the complete virus. This process, together with the solution of the structure of the flat doughnut-shaped protein disks by X-ray analysis, yielded a full description of the protein and RNA interaction, giving a great contribution to the understanding of how proteins and nucleic acids interact.
The general conclusion derived from the story of the tobacco mosaic virus assembly was that one must distinguish between the design of a structure and the construction process used to achieve it. As Klug himself emphasised in his later recollections, “although TMV looks like a helical crystal and its design lends itself to a picture of simple addition of subunits, its construction actually follows a more complex path that is highly controlled. It illustrates the point that function is inextricably linked with structure and shows how much can be done by one single protein. A most intricate structural mechanism has evolved to give the assembly an efficiency and purposefulness, and we now understand its basis. The general moral of all this is that not merely did nature once again confound our obvious preconceptions but that it also left enough clues for us to finally puzzle out what is happening. As Einstein once put it, 'Raffinert ist der Herr Gott, aber boshaft ist er nicht: Subtle is the Lord, but he is not malicious'.”
Crystallographic electron microscopy
When Klug's research group moved to Cambridge in 1962, they turned to electron microscopy because of the speed with which it enabled them to tackle new subjects, and also because it produced a direct image, or so they thought. They began their studies on viruses, both spherical and helical, but soon discovered the limitations of electron microscopy deriving from a series of different reasons related to the operating conditions of the microscope. When they were investigating viruses such as those that cause polio and warts in humans, such viruses appeared to be fuzzy spheres in conventional electron micrographs and could not be seen clearly enough to determine their structure accurately. Armed with a theory of virus design and data from X-ray crystallography, they had some ideas on how spherical shells of viruses might be constructed. They began to build models of viruses interpreting the different electron pictures through careful analysis, thinking that they would be able to see the fine detail in electron micrographs. But it came out that a fundamental problem was related to the large depth of focus (several thousand angstroms) of conventional microscopes, so that the image of all features along the direction of view was actually a two dimensional superposition of different levels in the three dimensional structure.
Up to that time, X-ray diffraction and electron microscopy had been largely complementary techniques for imaging biological structures. An electron microscope is a direct analogue of an optical microscope, using a beam of electrons in place of light and an electric or magnetic field in place of lenses. Since the wavelength associated with electrons is so much less than that of light, the resolving power of the electron microscope is hundreds of times that of an optical microscope. The electron microscope was useful for directly imaging structural features on a much wider variety of specimens, but also at a much lower resolution, only to a stage where the coarse outlines of the particles could be discerned, due to the relatively large size of biological molecules and the low scattering power of organic nuclei.
On the other hand, the technique of X-ray diffraction was used by crystallographers for high-resolution mapping of the structure of biological molecules, ranging from relatively simple amino acids to complex proteins, provided that they could be prepared in crystalline form. During diffraction experiments, X-ray radiation is scattered by the atoms present in the crystal and the intensity of the scattered X-rays are measured as reflections. However, each spot on a diffraction pattern provides information only about the intensity of the X-ray waves that have been deflected off the atoms in the crystal into the path of that spot. Ideally researchers also needed to know the phase of the wave, i.e. which particular point in the undulating cycle of movement from one wave peak to the next these X-ray waves are at when they hit the film and form each spot in the diffraction pattern. Various techniques for recovering this phasing information had been the focus of many efforts by many researchers. However, for several reasons many workers felt that they were not satisfactory as a direct means of solving more complex compounds.
Recognising the underlying physical and mathematical unity in the optics of image formation and diffraction of light, X-rays and electrons, Klug realised that both the amplitudes and the phase are present in electron microscopy images. The phase is preserved by the focusing of the diffracted electron beam into an image and could thus be extracted from its Fourier transform, a mathematical technique corresponding to forming the diffraction pattern that Klug knew about from crystallography.
The core of Klug's idea was that the Fourier transform of a two-dimensional projection of a three-dimensional object is identical with the corresponding central section of the three-dimensional transform of the object. An electron micrograph is essentially a projection of the specimen in the direction of view. In order to reconstruct a three-dimensional image of the specimen, it is necessary to be able to combine data from a number of different views. The three-dimensional transform could therefore be built up plane by plane by using the transforms of different projected views of the object. If sufficient data are available, the object can then be reconstructed by Fourier inversion of the resulting three-dimensional transform. The Fourier method has the advantage that because it is carried out in steps, i.e. formation of the two-dimensional transforms, and then recombination in three dimensions, it is possible to assess, select, and correct the data going into the final reconstruction. The final step was similar to the method which was used by crystallographers for building up a three dimensional map of the structure. The aim was to extract from electron micrographs the maximum amount of reliable information about the two- or three-dimensional structures that were being examined.
Generally, more than one view is necessary, but the method is most powerful for objects containing symmetrically arranged subunits, for there a single image effectively contains different views of the structure, reducing the number required. In principle, however, the method is applicable to any kind of structure, including individual, unsymmetrical particles, or sections of biological specimens.
It was the development of computer technology that dramatically extended the possibilities to process and analyse digital electron micrographs to reveal otherwise hidden information. In order to be able to perform operations such as Fourier transforming, the electron micrograph was first scanned with a computer-controlled film scanner providing a digitised image of the optical density distribution of any selected area of the micrograph. All further mathematical operations and the combining of different views were then performed with a digital computer.
The method of crystallographic - or Fourier - electron microscopy was announced by Klug and his talented new postdoc David DeRosier in Nature in early January 1968. They demonstrated that starting from a limited set of electron microscopy images, they could reconstruct the original structure in three dimensions. Particles with helical symmetry are the most straightforward to reconstruct, because a reconstruction can be made from a single view of the whole particle, to a limited resolution, set by the helix symmetry. In physical terms, this is because a single image of a helical particle presents many different views of the repeating subunit, and it was this simplification that led them to use the tail of bacteriophage T4, a common virus, as a first specimen to which they successfully applied this procedure for 3-D image reconstruction. It represented a major breakthrough in macromolecular structure determination. Three-dimensional reconstruction was immediately applied to actin filaments and became immensely valuable to structural cell biologists studying fibrous proteins, as it could be applied to membrane proteins and other structures of complex biological materials.
The principle of the three-dimensional reconstruction technique introduced by DeRosier and Klug created a revolution in structural molecular biology. Hundreds of macromolecular structures were determined by this method that also had a great impact on inorganic crystals and became the basis of the X-ray computer-aided tomography (CAT) scanner developed by Allan M. Cormack and Godfrey N. Hounsfield, who were jointly awarded the 1979 Nobel Prize in Physiology or Medicine.
Chromatin and nucleic acid-binding molecules
The work on viruses had given results not only of intrinsic interest. Klug's intensive work with the structure and functional organisation of the tobacco mosaic virus RNA-protein complex also exemplified his approach of chemically dissecting out parts of complex structures for detailed analysis by X-ray diffraction, and finally correlating such results with information about macromolecular organisation of the intact assembly from electron microscopy. The difficulties in tackling large molecular aggregates within studies connecting cellular and molecular levels of organisation led to the development of methods and techniques that could be applied to other systems.
In 1972, Klug began applying this procedure to the study of chromatin, the condensed compound of DNA and histones - special proteins found in eukaryotic cell nuclei - that makes up the chromosomes of higher organisms. The most important function of chromosomes is to carry genes - the functional units of heredity, segments of DNA that contain the instructions for making a particular protein or a set of closely related proteins. Each chromosome consists of a single, enormously long linear DNA molecule associated with proteins that fold and pack the fine DNA thread into a more compact structure. In addition to the proteins involved in packaging the DNA, chromosomes are also associated with many proteins required for the processes of gene expression, DNA replication, and DNA repair. We now know that each human cell contains approximately 2 meters of DNA if stretched end-to-end; yet the nucleus of a human cell, which contains the DNA, is only about 6 micrometres in diameter. This is geometrically equivalent to packing 40 km (24 miles) of extremely fine thread into a tennis ball! The complex task of packaging DNA is accomplished by specialised proteins that bind to and fold the DNA, generating a series of coils and loops that provide increasingly higher levels of organisation. It is the way in which DNA is tightly folded that actually allows it to easily become available to the many enzymes in the cell that replicate it, repair it, and use its genes to produce proteins.
In the early 1970s, when Klug's research group took up the study of chromatin, the aim was to try to understand the structural organisation of chromatin at various levels and to see what connections could be made with functional controls. At that time, protein chemists had shown that the protein component of chromatin consists largely of just five types of histones, and although they were known to be essential for organising DNA into compact structures in chromosomes, no structural details were known. Over the years 1972-1975 it was shown that four of the histones are responsible for the first level of structural organisation in chromatin. They fold successive segments of the DNA about 200 base pairs long into compact bodies of about 100 angstrom in diameter. Different lines of evidence previously obtained by different researchers had provided biochemical indication for a regular basic organisation of chromatin, but it was Roger Kornberg, a postdoctoral fellow working with Klug and Crick at the Medical Research Council, who identified in 1974 the basic chromatin subunit that was named “nucleosome”. The discovery of the nucleosome, the fundamental unit for the modulation of chromatin function, revolutionised the perception of DNA and DNA-based processes, such as transcription, replication and repair. In higher organisms the substrate for the expression of genes is not naked DNA, but chromatin in which DNA is packaged in nucleosomes, so there are complex mechanisms for making the control regions accessible to the transcription machinery. Chromatin was too large a molecular aggregate to allow a direct structural determination even by Klug's methods. With his co-workers, Klug, however, succeeded in breaking down chromatin to fragments that were small enough to be studied by X-ray diffraction and electron microscopy. The nucleosome, in being the fundamental repeating subunit of chromatin, gave the first level of higher-order packaging of chromosomal DNA by histones. In 1976, Klug and Finch established another level in the hierarchy of DNA packaging. They proposed the “solenoidal” model for winding nucleosomes into the next level of organisation showing that the nucleosome filament is coiled into yet another helix, the “solenoid.” They demonstrated that the fifth histone is necessary to produce this further compaction required in chromosomes. Crystals of the nucleosome core were gradually improved and the group, then including Tim Richmond, solved its structure to 7-angstrom resolution in 1984. They provided the first evidence that the histone core, consisting of an octamer of pairs of four histones, wraps DNA around itself in about two turns of a left-handed superhelix. Each nucleosome is wrapped around a histone protein core, leading to an approximate sixfold-length compaction of the DNA. The histones themselves are gathered into coils so tight that a single strand of human DNA, which is about two meters in length, fits within the nucleus of the cell, which is less than a hundredth of a millimetre in diameter. The manner in which this folding is achieved, genes organised and their expression controlled, developed as the subject of intense study throughout the world. In addition to compacting the DNA, the histone octamer plays a key role in the transcription of the DNA surrounding it. In 1997 Richmond, who had in the meantime set up his own laboratory at ETH, Zurich, took the analysis to 3-angstrom resolution, revealing the interaction between the histone molecules and DNA in atomic detail. The study of the compacted association of histones and DNA within chromatin structure has revealed its major impact on the regulation of gene expression and other protein-DNA transactions. During the last years, these investigations are more and more providing the details of the complex chromatin dynamics and the clues to the structural control of the reading of the genetic message in DNA.
In 1985, Klug was also the discoverer of zinc-finger proteins, an independently folded protein module that binds specific DNA sequences by means of arrays of “fingers”. If a gene is to be activated, proteins known as transcription factors, attach themselves to a segment of the gene called the promoter. This assembly forms a kind of “on switch”: it enables an enzyme to transcribe a second genetic segment from DNA into RNA. In most cases, the resulting RNA molecule serves as a template for the synthesis of a specific protein, or string of amino acids; sometimes RNA itself is the final product. Yet scientists had continued to wonder exactly how a transcription factor picks out its particular docking site on a promoter, distinguishing that site from the masses of other DNA found in a cell. It turned out that many transcription factors included the small projections called zinc fingers that are perfectly suited to DNA recognition. Their role is to select and grip specific binding sites on DNA, enabling enzymes to transcribe. Since then these interaction modules have proven ubiquitous in the biological world and the name has now come to encompass a wide variety of differing protein structures. The modular nature of these proteins has made it possible to design synthetic proteins with a wide range of specificities, opening the prospect of targeted therapies for a wide range of diseases.
Aaron Klug was awarded the Nobel Prize in Chemistry in 1982, “for his development of crystallographic electron microscopy and his structural elucidation of biologically important nucleic acid-protein complexes,” the only Nobel Prize on electron crystallography. By that time, his successful style of bringing together results using a variety of techniques had deeply changed the way in which complex biological structures are visualised and interpreted. In 1986, he was appointed the director of the Laboratory of Molecular Biology, which he had helped to develop into a lab world-renowned for its excellence, and was President of the Royal Society from 1995 to 2000, when he returned to full-time research investigating the structure of DNA and RNA binding proteins regulating gene expression and continuing his studies on the zinc finger proteins and their interaction with DNA.
Bibliography
Amos L. A. and Finch J. T. (2004) Aaron Klug and the revolution in biomolecular structure determination. Trends in Cell Biology 14 (3): 148-152
Barker G. R. (1993) Aaron Klugn. In Laylin K. J. Ed. In Nobel Laureates in Chemistry 1901-1992 (American Chemical Society and the Chemical Heritage Foundation), pp. 654-659
Caspar D. L. D. and D. J. DeRosier (November 12, 1982) The 1982 Nobel Prize in Chemistry. Science 218 (4573): 653-655
Kornberg R. D., Klug A. (1981) The Nucleosome. Scientific American 244 (2): 52-64
Jonathan P., Butler G., Klug A. (1978) The Assembly of a Virus. Scientific American 239 (5): 62-69
Klug A. (1982) From Macromolecules to Biological Assemblies. Nobel Lecture,
http://www.nobelprize.org/nobel_prizes/chemistry/laureates/1982/klug-lecture.html
Klug A., Rhodes D. (1993) Zinc Fingers. Scientific American 293 (February 1): 56-65
Klug A. (1999) The tobacco mosaic virus particle: structure and assembly. Philosophical Transactions: Biological Sciences 354 (1383): 531-535
Klug, A. (2010) From virus structure to chromatin: X-ray diffraction to three-dimensional electron microscopy. Annual Review of Biochemistry 79: 1-35
Wasson T. (ed.) (1987) Klug, Aaron. In Nobel Prize Winners, H. W. Wilson Company, New York, pp. 560-562