Prof. Dr. Masatoshi Koshiba > Research Profile
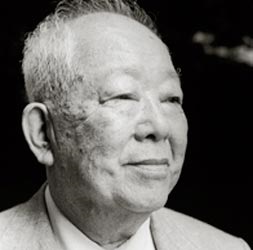
by Luisa Bonolis
Masatoshi Koshiba
Nobel Prize for Physics in 2002 together with Raymond Davis Jr. and Riccardo Giacconi "for pioneering contributions to astrophysics, in particular for the detection of cosmic neutrinos".
From Tokyo to Chicago University and Back: Cosmic Rays and Accelerators
Masatoshi Koshiba was born in 1926 in Toyohashi, a city near Nagoya, Japan. His father was an army officer, so when Masatoshi was 13, he had to enter the military school during the war. However, one month before the entrance examination, he got polio, which made his right arm numb, making him exempt from military service during the war. As he could not do anything athletic, his hobby at the time was to build from scratch model airplanes, made with bamboo and powered by a rubber band. He was seriously thinking of becoming a model airplane shop owner, but at the same time he liked mathematics and during his illness he read a small book, which was a dialogue with Albert Einstein. Even if he did not understand the simple statements contained in the book, they did attract his attention. In 1945, four months before the end of World War II, he entered high school. At that time he was in Tokyo, which was devastated by bombing. Also the area around his school was pretty much burned down. At that time, Koshiba was quite interested in literature, seriously thinking of entering the German literature department in the university. But by chance he heard one of his professors saying that he could not choose the physics department, but rather German literature or Indian philosophy. This statement made Koshiba furious, so he started studying physics and after one full month of concentrated work he passed the physics department requirement. However, as his family had money difficulties, while attending the university he worked hard during the evening and night, doing all sorts of odd jobs, in order to maintain himself and help his family. Because of his not being able to attend courses, as he was nearing graduation, his records were very poor, and therefore, asking for a scholarship in the graduate school or an assistantship was out of the question for him. Luckily, he was helped by a well-known philosopher called Amano, who knew the physicist Sin-Itiro Tomonaga and gave Koshiba a letter of introduction. Tomonaga, who had worked in Leipzig in the group of Werner Heisenberg, had returned to Japan at the outbreak of the war. After finishing his doctoral degree on nuclear physics, he was appointed a professor at the Tokyo University of Education. For his contributions to the development of quantum electrodynamics, he would be later jointly awarded the Nobel Prize in Physics in 1965 along with Richard Feynman and Julian Schwinger.
Koshiba spent two years in the graduate school of the University of Tokyo. One of his friends, who was a research assistant of the department, suggested him to consider experimental physics, as, he said, the competition in theoretical physics was very severe. He then described a new type of nuclear emulsion, invented by Cecil Powell's Bristol group in England. Koshiba and his friend obtained a small sample of this Ilford nuclear nuclear emulsion and started a small experiment by exposing it on top of Mt. Fuji. Using such nuclear emulsions, Powell, Cesare Lattes and Giuseppe Occhialini had been able in 1947 to demonstrate the existence of the pion, a particle that turned out to be an example of the mesons predicted by Hideki Yukawa in 1935 as the carrier particles of the strong nuclear force between protons and neutrons. The discovery of the pion had also clarified the nature of the muon, a very penetrating particle, which finally turned out to be a product of the decay of the pion, too weakly interacting to be a Yukawa particle as it had been for a long time erroneously hypothesised.
In 1949, Yukawa, who had just become a professor at Columbia University, was awarded the Nobel Prize in Physics "for his prediction of the existence of mesons on the basis of theoretical work on nuclear forces", thus becoming the first Japanese Nobel laureate. At that time, Yukawa had met Robert Marshak at a physics conference in New York. Marshak wanted to get a good student from outside the country, and Yukawa recommended Koshiba, who was thus able to become research assistant at Rochester University in 1953. Because of his lack of training in experimental techniques at the University of Tokyo, he was required to take laboratory courses. Because of the war, Japan had in fact very few laboratory facilities, and all the accelerator-related equipment had to be destroyed, so all Japanese students who went to Rochester had to take such courses to improve their technical training. Very soon he was able to pass the qualifying exams and began to work with Mort F. Kaplon with nuclear emulsions that were flown on balloons. For his thesis topic, he chose ultra-high-energy phenomena in cosmic radiation. He received his doctoral degree in April 1954, and at the beginning of July he was offered a research associateship by Marcel Schein of the University of Chicago. Schein was a cosmic ray physicist who had shown in 1940, using balloon flights, that the primary cosmic rays were mostly protons. As his first work, Koshiba was given balloon-exposed stacks to analyse in order to determine the chemical abundances in the primary cosmic radiation. In those days the interest of primary cosmic ray researchers was concentrated on the relative abundance of light nuclei, lithium, beryllium and boron, as compared with the abundances of CNO. This was an important quantity to make estimates of the amount of interstellar material the primary cosmic radiation had to traverse before reaching the detectors.
Koshiba discussed his work with the great astrophysicist Subramanyan Chandrasekhar, who would be awarded the Nobel Prize in Physics 1983 “for his theoretical studies of the physical processes of importance to the structure and evolution of the stars”. Chandrasekhar explained to him the difference between different types of stars, so that Koshiba concluded his paper on the matter by saying that judging from the observed chemical abundances of primary cosmic radiation, a likely ion source for the primary radiation could be supernovae, and he thanked Chandrasekhar at the end of the paper. Many people had speculated about the idea that supernovae could be the sources of cosmic rays, but Koshiba was showing this possibility in the form of experimental data.
After working about three years in Chicago as a research associate, in 1957 Koshiba was offered an associate professorship at the Institute for Nuclear Study at the University of Tokyo. But in 1959, Schein wrote to him, saying that he was now organising a very large-scale international collaboration using a big stack. He wanted Koshiba to help him in this international enterprise. So he took a leave of absence from the University of Tokyo. But three months after his arrival, Schein suddenly died and the University did not know what to do. So they asked Giuseppe Occhialini, a well-known cosmic-ray physicists, who was at that time spending a year in Bruno Rossi's group at MIT, to come and give advice. After interviewing all of Schein's group members, Occhialini recommended Koshiba as the one to succeed Schein and to restart the program. With the help of Occhialini, who went to Chicago almost every month, Koshiba got the research funding and started to go on exposing the remaining stack. About 12 countries were involved in the project and Koshiba officially acted as director of the laboratory for high-energy physics and cosmic radiation of the University of Chicago. In early summer 1960, a big balloon was launched, but the receiving circuit of the balloon was damaged by lightning, so that this expedition was unsuccessful. A new balloon was launched that reached an altitude of about 30 km. The most relevant result was the observation of a nuclear interaction in the 1000 GeV energy range, which at the time was still unavailable from accelerators. At the time, new powerful accelerators had widely superseded cosmic rays as source of beams of well-collimated high-energy particles, and cosmic ray physicists were now moving to the study of rare ultra-high energy events.
Koshiba remained in Chicago until the end of August 1962, then he returned to Tokyo and after one year he left the Institute for Nuclear Study and moved to the Physics department of the Faculty of Science as associate professor. He had just a block of nuclear emulsion to give to his graduate students and thus he worried about their future. If they only learned the nuclear emulsion technique, it would be not easy for them to find jobs. For this reason he thought of switching to the analysis of bubble chamber pictures. But he was rather discouraged because in the end, he thought, one could still be “only one of the collaborating groups of a big bubble chamber collaboration”. There were no good accelerators in Japan in those days and so he thought of continuing to do cosmic rays, but with electronics counters. One of the results obtained from the analysis of the 1000 GeV nuclear interaction found in the big nuclear stack was that there was good reason to expect multiple muon events to be observed deep underground. Muons have a great penetrating power, so that Koshiba was expecting a more abundant occurrence of such multiple muon phenomena. There was already a report of such events from a Russian and from a US group. So, as a first electronic attempt, Koshiba set up an underground experiment in 1969.
He contacted the Kamioka Mine Company and explained his ideas to the president. By order of the president himself, their experimental cave was prepared, and telephone and power lines were installed at the company's expenses. Koshiba set up a rather large, 3 by 3 meter, spark chamber, which could be used to follow the tracks of charged particles. They triggered this spark chamber by two layers of plastic scintillators. The experiment was successful and they did observe multiple muons up to a multiplicity of 18. It was this experiment by which Yoji Totsuka received his Ph.D. After several years at DESY in Germany, he would eventually become director of the Kamioka Observatory and have a leading role in the Super-Kamiokande collaboration that provided the first definitive evidence for neutrino oscillations. But this breakthrough was still far in the future. Similar experiments on muons, much bigger in size, were being performed in Italy by Barry Barish, under the Gran Sasso mountain. They, too, observed similar multiple muon events. But these were rather complicated phenomena, difficult to interpret, and not many people were interested in them.
In the meantime, Koshiba was making plans for the future. During a conference in Moscow in 1960, he met Andrey Budker, the great Soviet physicist, best known for his invention of electron cooling. Budker, who was with Bruno Touschek a pioneer of electron-positron colliding machines, asked Koshiba to go to Novosibirsk, where he was building such a machine, and carry out a joint experiment. But Budker was stricken by an illness of the heart, and in the meantime Koshiba decided to go to Frascati, Italy, where the first electron-positron collider AdA had been built by Touschek and his collaborators, and now the bigger machine Adone, was working. A number of experiments had already been done, but the upper limit of the energy achieved was only 3 GeV. Then Koshiba went to CERN, where a proton-proton colliding machine was working, but he was not interested in hadronic collisions, which are much more complex than electron-positron collisions. So after CERN, Koshiba went to DESY, in Hamburg, where there was a big electron synchrotron and where DORIS, a new electron-positron collider was being built. There were two experiments being prepared, so Koshiba and his group joined one of the two, called DASP, for Double Arm Spectrometer. Four young researchers of Koshiba's group participated in the experiments. As Koshiba himself recalled, at that time many people thought that nothing new would come out of having just the electromagnetic interaction. However, only a few months after they started the DASP operation, there was the parallel discovery by the groups at Stanford and at Brookhaven of the J/Psi particle, which opened a new era of particle physics. So the DESY group immediately changed the beam energy and followed up, making some novel discoveries of their own. Koshiba was very happy to have sent his most advanced young people to work within such an international collaboration.
In the meantime, DESY built a bigger electron-positron colliding machine, called PETRA. Koshiba joined Joachim Heintze's group based in Heidelberg, and together they built a new kind of detector - originally Koshiba's idea - the lead-glass electromagnetic shower detector, designed to cover large solid angles so that it would not miss any interesting events. They organized an international collaboration JADE, which is an acronym for Japan, Deutschland, and England, the three countries from which the participating universities originated. In later years an American group participated, giving the “A”. JADE operated from 1979 to 1986. Its most important scientific achievement was the discovery of the gluon in three jet events. It also helped greatly in establishing quantum chromodynamics. The JADE jet chamber is now exhibited in the physics lecture hall at the University of Heidelberg.
But Koshiba wanted to have something besides this big international collaboration, over which he could have complete control. Moreover, he felt that all of those accelerator experiments were becoming too routine. In order to attract the attention of younger people he thought they needed something different, that is some experiment in the country where he could use and train young graduate students and undergraduates too.
The Proton Decay
In fall of 1978, Koshiba received a telephone call from Hirotaka Sugawara, the head of the theoretical division at the National Laboratory for High Energy Physics. Sugawara proposed him to do an experiment testing the Grand Unified Theory - which is a theory combining strong forces, weak forces, and electromagnetic forces - in particular the minimal one based on the symmetry SU(5), regarded as the prototype of such theoretical attempts. The minimal SU(5) model predicted a proton decay partial lifetime of 3x1027 to 6x1031 years for the most favoured decay mode, that is a proton decaying into positron and neutral pion. This decay mode implies that the rest mass of the decaying proton is completely converted into electromagnetic shower energy, about half of which goes in one direction and the other in the opposite direction, because the neutral pion decays quickly into 2 photons.
Koshiba immediately remembered having discussed with Occhialini the use of a deep underground lake for shielding the photomultipliers. They had stored their big stack in a salt mine cave near Cleveland, in order to shield it against cosmic rays. Koshiba had remarked thta since it was a salt mine, if one made a pool-size hole in the ground and poured water in it, one would get an underground lake of saturated salt water. After a few days, the dirt would settle to the bottom and one could get very clear, transparent salt water. Being saturated, there would be no bacteria or plant growth; it would stay very clean and transparent for years. He had wondered what they would observe if they could install a large number of photomultipliers facing downwards. In media like water, the light velocity is reduced to three-quarters the vacuum value. Therefore, when a particle is traversing water with very high energy, its velocity can exceed the velocity of light in the water and it generates a sort of light boom, a coherent cone of blue light know as Cherenkov radiation. The cone is emitted with the axis on the trajectory of the moving electrically charged particle and with an opening angle that is determined by the velocity of the particle and the index of refraction of the medium. As cosmic ray muons do come down to that depth, the Cherenkov light they produce would thus be pointing downwards. So if one had photomultipliers on the surface facing downward, one would not see those downward-going muons. One would observe just some scattered light. In the early 1960s, the photomultiplier was a very small, expensive and not very dependable detector and Koshiba and Occhialini did not go further with this idea; but when Koshiba received the call from Sugawara, it was immediately clear to him that the most favoured mode for proton decay, into positron and neutral pion, and then neutral pion into two gamma rays, was an ideal decay channel for water Cherenkov detectors, producing an easily recognisable three-ring signature. The Cherenkov light produced by the different particles is in fact reconstructed as a ring of hit photomultiplier tubes. The appearance of the ring can be used to identify the originating particle. Therefore, having a photosensitive device on the inner surface, one would observe this Cherenkov light immediately, and there would be no question about the nature of the event. However, unless one had a sizeable fraction of the entire enclosing surface covered by photocathodes, one could not hope for a detailed study of nucleon decays into various modes. So within about an hour after the phone call from Sugawara, Koshiba took a piece of paper, drew the detector and photomultiplier installation. By December 1978, Koshiba's proposal was approved, and they immediately started work. The next month, in January 1979, Koshiba received a copy of the Irvine-Michigan-Brookhaven (IMB) project for a proton decay detector. Koshiba had to start thinking very seriously, since the US scientists were planning a much bigger detector. And they had much more money. How could they compete in the proton decay work with IMB? If their aim was only to detect a proton decaying into a positron and a neutral pion they did not have a chance, because IMB had a much bigger volume and they would have no difficulty to identify such a decay. Koshiba thought that there was the possibility of observing other decay modes. But in order to detect such other possible decay modes, they had to use very sensitive detectors. Ideally, the entire inner surface would be covered by photocathode. But how could one approach that limit with the limited amount of funding? Koshiba came up with the idea of developing very large phototubes. Then, using the same number of channels, say one thousand, these big phototubes would give much more sensitivity than the smaller ones.
They had previous experience with RCA, the Radio Corporation of America, an electronic company that had built thousands of phototubes for the DASP and JADE experiments. Koshiba asked them to try producing 20-inch phototubes. The company actually had experience with tubes up to something like 12-inches diameter and were reluctant to go beyond anything bigger than 14 inches in diameter. But Koshiba insisted on the 20 inches; that was the size he really wanted. One problem was the degree of vacuum; more important was the fact that the phototubes had to be immersed in deep water, which meant additional pressure, so the glass had to be of the correct thickness to withstand the pressure differences. Further problems arose from the necessity of avoiding different arrival times of photoelectrons from various parts of the photocathode through a careful design of the shape of the photocathode and the shape of dynode. They needed extremely precise timing so that Koshiba sent a couple of his assistants to help design and test the tube.
They did not have any salt mines, so they had to dig a new cave, and all this preliminary work required new funds that Koshiba successfully obtained. He chose the Kamioka mine because he already knew the site and had experience with the company for many years, dating from his first underground muon experiment. The people in the mining company made a test hole and examined the composition of the rock; also the shape of the cave had to be taken into account. Upon the advice of a professor in the engineering faculty who was an expert on this kind of things, they decided on the shape of the cave. Luckily, the cave was located in the oldest rock formation on the entire island. It was very old, very solid rock, necessitating the use of explosives; a jackhammer would not do. There had been mining in that area for over one thousand years. The miners there were very experienced. There can be a lot of dangerous situations in a deep mine.
The tank was planned to lie beneath 1 kilometre of earth and rock, which would screen out the expected background from cosmic ray muons, which would confound its photomultiplier tubes. They lined the inner side of the tank with a coating to prevent water from leaking. Originally, their aim was to search for proton decays. For that purpose, they didn't need any anti-coincidence system, because the signal was large and very typical, that is back-to-back. So they did not worry about the background. The detector was a cylindrical tank containing 3000 tons of pure water and had about 1000 photomultiplier tubes attached to the inner surface. They installed the tank and laid down the phototubes on the wall and on the bottom and top and filled it with filtered water. The Kamioka experiment was named KamiokaNDE for Kamioka Nucleon Decay Experiment. To observe the decay of a particle with a lifetime as long as a proton an experiment must run for a long time and observe an enormous number of protons. Water can detect relativistic charged particles through their production of Cherenkov radiation. The distinct pattern produced allows for particle identification. In early July 1983, Kamiokande's detector started taking data. It performed successfully for the original aim of searching for nucleon decays into various modes, but it failed to observe proton decay and set what was then the world's best limit on the lifetime of the proton. Later, the upgraded version of the experiment, Kamiokande-II, continued the search for proton decay and again failed to observe it, but the experiment again set a lower-bound on the half-life of the proton. If protons decay at all, they did not decay as quickly as some theories predicted. After both IMB and Kamiokande did not find the event, the experimentalists pushed the lifetime up to 1033 years.
Solar Neutrinos
At the very beginning, when it was only a matter of finding positrons and neutral pions as the most favoured decay mode of the proton, IMB had very much of an advantage over Kamioka. But even if IMB discovered the decay, eventually the Kamioka detector would supplement that information by observing other types of decay modes. But the Kamioka detector would find its true purpose elsewhere.
Already in the original proposal, Koshiba had mentioned the detection of solar neutrinos. Unlike the photons emitted by the Sun, neutrinos produced in the various fusion chains are of particular interest because they probe the interior of the Sun and thereby are a sensitive test of the standard solar model. In several of the nuclear beta-decay or electron-capture reactions, electron-type neutrinos are emitted, whose energies extend to approximately 14 MeV. However, the main flux of neutrinos comes from the proton-proton interaction, but they have a low energy, only up to 420 keV. The possibility of observing solar neutrinos began in fact to be discussed seriously when it became clear that the boron-8 might be produced in the Sun in sufficient quantities from beryllium-7 and protons to produce an observable flux of high-energy neutrinos from boron-8 beta decay.
Koshiba was aware that, according to the Standard Solar Model, the boron-8 decay, a rare nuclear reaction in the solar energy production cycle, produces only about 0,02% of the solar neutrinos, but these few neutrinos are produced with a continuous spectrum of energies that extends to 14 MeV. These are much larger energies than those of neutrinos coming directly from the proton-proton chain. The idea was that when boron-8 neutrinos are scattered off of atomic electrons in the water, the recoil electron direction would be oriented along the direction of neutrino travel and the electron would make a weak Cherenkov ring in the detector.
For many years after Pauli proposed the existence of a neutral particle of spin 1/2 emitted alongside the electron in beta decay, it appeared that such elusive particle would never be detected, even if the success of Fermi's theory of beta decay incorporating Pauli's ``little neutral one'' firmly established the existence of the neutrino in the eyes of nuclear and particle physicists. The smallness of cross section made the neutrinos still a hypothesis; many physicists thought that neutrino detection was almost impossible. The advent of nuclear fission in the 1930s and 1940s offered an unprecedentedly intense source of neutrinos, which for the first time made the experimental detection of these elusive particles a realistic proposition. In 1945, Bruno Pontecorvo proposed to reveal free neutrinos coming from reactors through the radiochemical method. The basic idea was to use chlorine as a target and neutrino detector. Upon interaction with an electron neutrino, a chlorine-37 atom transforms by inverse beta decay into a radioactive isotope of argon-37, which can then be extracted and counted in a small gas counter detecting its decays. In such a way, one would be able to determine how many neutrinos had been captured. But at that time it was not clear that neutrinos coming from the processes taking place in nuclear reactors are anti-neutrinos, which could not trigger Pontecorvo's radiochemical reaction. The neutrino was then revealed using a different process by Cowan and Reines in 1956. By that time, thanks to many years of technological and scientific developments, it was possible to think about detecting reactions triggered by neutrinos. Using the newly developed technology of organic liquid scintillators, Clyde Cowan and Fred Reines were able to detect anti-neutrinos from the intense beam of a powerful nuclear reactor. The reaction they used was the inverse of neutron decay, in which a neutrino hitting a proton produces a positron and a neutron. The positron and the photons emitted in its annihilation were detected by the scintillation light together with the neutron's nuclear absorption photon in delayed coincidence. The neutrino had finally been observed, but the Nobel Prize for this achievement was awarded only in 1995, when Cowan was unfortunately no longer alive.
In 1956, there were two known charged leptons, the electron and the muon. The third lepton, the tau, was not discovered until 1975. Each of these had its antiparticle with opposite electric charge. Therefore, following the experimental observation of the neutrino, two immediate questions were: Is the neutrino distinguishable from its antiparticle? Is the neutrino produced in beta decay different from the neutrino produced in association with the muon in pion decay?
The first question was answered by Raymond Davis, who took up Pontecorvo's idea of the chlorine-37 reaction triggered by neutrinos. According to the law of lepton number conservation, which states that the total number of leptons minus the total number of antileptons is always constant, this reaction must involve a neutrino (not an antineutrino); in contrast, beta decay must involve an antineutrino (because an anti-lepton must be produced to balance the electron). Therefore, if the neutrino and antineutrino are different, reactor neutrinos would not convert chlorine-37 to argon-37. Ray Davis, later to become famous for his work on solar neutrinos, investigated this process using carbon tetrachloride (CCl4) as the target and the Brookhaven research reactor as the source. Over the period 1955-1960 he was able to show that the probability of this reaction was less than 10% of that expected on the assumption that the neutrino and the antineutrino were identical. This work, complementing Cowan and Reines' observation of neutrinos from a nuclear reactor, indicated that the neutrino and antineutrino were different particles, and that the law of lepton number conservation was obeyed in weak interactions. The second question was answered by investigating whether the neutrinos produced in pion decays, which are always associated with a muon (the pion decays into a muon + neutrino, never into an electron + neutrino) can subsequently be converted into electrons. If they can, then the two types of neutrino are not distinct. The experiment had been suggested by Pontecorvo in 1959. He proposed to test for any difference between electron-neutrinos and muon-neutrinos by using neutrinos that are emitted along with muons in the decays of pions to bombard a detector similar to that used by Cowan and Reines and then count how many electrons were produced in the inverse reactions in which the neutrinos were absorbed. No inverse reaction would occur if the muon-neutrino was indeed different from the neutrino that accompanied the electron in beta-decay, as it was suspected. The accelerator that Pontecorvo was planning was never built in the USSR, where he was living since 1950. But in New York, Melvin Schwartz conceived a similar idea and the experiment was carried out at Brookhaven in 1962. The pions produced in the bombardment of a beryllium target by 30 GeV protons from Brookhaven Alternating Gradient Synchrotron were allowed to decay and the resulting high energy accompanying muons were stopped by a thick iron absorber. Only the neutrinos associated with the muon in the pion-muon decay penetrated the absorber to reach the detector. By observing that the neutrinos from pion-muon decays were later able to produce only muons but not electrons, they concluded that muon-neutrino and electron-neutrino are different neutrinos. Leon Lederman, Jack Steinberger and Melvin Schwarz were awarded the Nobel Prize in 1988 for demonstrating the doublet nature of leptons through the discovery of the muon neutrino.
Then, in 1964, Raymond Davis and the theorist John Bahcall published back-to-back papers arguing that a 100000-gallon detector of perchlorethylene could be built, which would measure the solar neutrino capture rate on chlorine. Now the motivation was to use neutrinos to look into the interior of the Sun and thereby test directly the theory of stellar evolution and nuclear energy generation in stars. A great deal has been learnt about our Universe through the long history of optical astrophysics, e.g. the universal expansion, the chemical abundances, etc. The post-war development of radio-astronomy has shown, among others, the existence of the cosmic microwave background at 2.7 K, thereby making the Big Bang origin of our Universe quite plausible. X-ray astronomy has provided evidence for neutron stars and black holes. Today the Universe is observed across the whole spectrum, from radio through submillimeter and infrared to high-energy gamma-rays. However, all electromagnetic signals are modified strongly due to interaction with matter. This means that only information from the thin surfaces of hot stellar objects and from diffuse gaseous objects at rather high temperature can be conveyed by these signals. In order to probe the deep interior of a stellar object, be it a star or a galaxy, it is clear that a much more penetrating signal interacting with matter only through the weak force is to be used. The simultaneously emitted photons entirely lose their memory through the emission and absorption processes on their 10-million years journey to the surface.
In a cavity excavated in the Homestake Gold Mine in South Dakota, Davis placed 1478 meters underground a 380 cubic meter (100,000 gallon) tank of perchloroethylene, a common dry-cleaning fluid rich in chlorine. A big target deep underground was needed to prevent interference from cosmic rays, taking into account the very small probability of a successful neutrino capture, and, therefore, very low event rate even with the huge mass of the target. The argon-37 nuclei produced upon interaction of solar neutrinos with chlorine-37 were extracted from the liquid every few months, comparable to the decay lifetime of argon-37, by helium gas flushing and subsequent additional processes. The purified argon atoms were introduced into a specially designed proportional counter. The detection of the 2.8 keV Auger electrons accompanying the electron capture of argon-37 (back to chlorine-37) with a half-life of 35 days constitutes the signal of the experiment. In this way, Davis was able to determine how many neutrinos had been captured.
Ray Davis's Homestake Experiment was the first to detect solar neutrinos, strong evidence that the nuclear theory of the sun as hypothesised by Hans Bethe and Carl Friedrich von Weizsäcker in the 1930s was correct. The Sun shines by converting hydrogen into helium deep in its interior. According to this theory, four protons - hydrogen nuclei - are changed into a helium nucleus, two positrons and two neutrinos, a reaction releasing a lot of energy. Because they interact so rarely, neutrinos can easily escape from the solar interior where they are created and bring direct information about the solar fusion reactions to the Earth. There are three known types of neutrinos. Nuclear fusion in the Sun produces only neutrinos that are associated with electrons, the so-called electron-neutrinos. The two other types of neutrinos, muon neutrinos and tau neutrinos, are produced in different processes occurring in laboratory accelerators or in exploding stars together with heavier versions of the electron, the muon and the tau.
In 1956/1957, rumours had reached Pontecorvo that Davis had observed a reaction triggered by antineutrinos from a nuclear reaction in his chlorine experiment. He thus suggested that these events could be due to transitions of reactor antineutrinos into right-handed neutrinos on the way from the reactor to the detector and published a first paper dedicated to neutrino oscillations in 1957. The concept of oscillation, which he had drawn from the behaviour of K particles, remained in Pontecorvo's mind and in several papers from 1957 to 1967 he anticipated the phenomenon of the deficit of the solar neutrinos due to the disappearance of electron-neutrinos and their transition to a different state during their travel to the Earth.
The first results from the chlorine experiments with solar neutrinos were published in 1968. Davis found figures consistently very close to one-third of what Bahcall had calculated. The first reaction from the scientific community was that either Bahcall or Davis had made a mistake. Models used to predict the rate of solar neutrino production are complex but make only a few assumptions - that the sun is powered by nuclear reactions that change the element abundances, that this power creates an outward pressure balanced by the inward pull of gravity, and that energy is transported by photons and convection. Bahcall repeatedly checked his calculations. The solar models continued to predict neutrino fluxes that exceeded measurements. Davis, too, insisted that there was nothing wrong with his experiment.
One year after the first chlorine results were published, Vladimir Gribov and Bruno Pontecorvo proposed that the explanation of the solar neutrino problem was that electron-neutrinos initially produced in the sun might be partially converted into other states, which could not produce signals in the chlorine-37 experiment of Davis. But in those days, this explanation was widely disbelieved by nearly all the particle physicists. The discrepancy between the standard solar model predictions and the chlorine observations became widely known as “the solar neutrino problem”. Very few people worked on solar neutrinos during the period 1968-1988. Davis's chlorine experiment remained the only one providing data on solar neutrinos in these two decades.
Koshiba of course knew about the Davis experiments for the detection of solar neutrinos, going on since the early 1960s. As he himself recalled in his Nobel Lecture, Davis's radiochemical work “could be considered as the conception of neutrino astrophysics and was in fact the impetus for us to begin seriously working on the solar neutrinos.” During the 1960s, Bahcall had also investigated the properties of neutrino electron scattering and showed that the forward peaking from boron-8 neutrinos is large, a feature that Koshiba incorporated two and half decades later in the Kamiokande experiment.
The enormous volume possible in a water Cherenkov detector could overcome, like in Davis's experiment with perchloroethylene, the problem of the very small cross section of the 5-14 MeV solar neutrinos Koshiba wanted to reveal. Water, too, is an inexpensive material, acting as the target/sensor in a very large detector. However, water Cherenkov detectors offered the further possibility of studying individual neutrino-electron interaction candidate events on an event-by-event basis, while month-to-month observations were required in the radiochemical experiments, where argon-37 atoms, resulting from the interaction between chlorine-37 and neutrinos, were collected only after a certain number had accumulated in the tank. In using a different target, interaction process, detector technology, the water Cherenkov Kamiokande experiment would be a very complementary test of Davis's results.
Of course, in order to be sensitive to the lower energy events expected from solar neutrinos, much depended on how much one could reduce the background. The possibility of observing solar boron-8 neutrinos by neutrino-electron elastic scattering was attractive enough to justify considerable additional efforts in this direction. At the time, it was already well known that there were deviations from the prediction of the standard solar model. Only one-third of the predicted value was observed. Koshiba decided that there was another use for his instrument, but he needed to install additional anti-counter layers and to improve the event reconstruction using a timing device. The real problem was that he did not have money to buy one thousand channels of TDC. During an international conference in the US in 1984, Koshiba convinced Alfred Mann, of the University of Pennsylvania, that it was a good idea to try and observe solar neutrinos through electron scattering. A new collaboration was started and Mann's group took care of preparing the timing devices and installing them. Kamiokande-I was dismantled, and Kamiokande-II started taking solar neutrino data from early January 1986. A great difficulty in this kind of experiment was reducing the background effects, mainly those due
to the presence of radon, a gas occurring naturally in the environment as a result of the decay of radioactive elements in the soil and contributing to environmental radioactivity. They had to make the whole system of the detector airtight, so that the atmospheric radon didn't come into the detector. In order to see the signal of the solar neutrino, you had to go through various stages of background reduction, and in order to do that you had to accumulate a certain number of events. As always, the signal was masked by the background. For many months they worked in a pure data-collecting mode and in late 1986 they completed their revisions to make the detector sensitive to solar neutrinos.
Then, something happened on February 23, 1987. Like the Sun, all stars emit neutrinos, but the extragalactic neutrinos that swarmed through the Earth on that day marked a special event. Some 170 million years ago, in a nearby galaxy called the Large Magellanic Cloud, a star nearly 20 times the size of the Sun died in a catastrophic explosion, flinging subatomic debris out into space. In 1987, the first signals from the catastrophic event reached the Earth: myriads of neutrinos bearing witness to the death of the distant star. The Kamiokande-II experiment happened to be running; the detector was online and taking data. Supernova and solar neutrinos have similar energies, much less than the energies that are relevant for proton decay. With the upgrades that had taken place the detector was sensitive enough to detect the thermal neutrinos produced by Supernova 1987A. When the data arrived in Tokyo to be processed, it was discovered that Kamiokande-II had recorded signs of 11 neutrinos. About three hours later, astronomers in the Southern Hemisphere saw the first light from the explosion, heralding the appearance of SN 1987A, the first supernova to be visible to the naked eye since Kepler's supernova in 1604, i.e., just a few years before the use, by Galileo, of a telescope for astronomical observation. After learning about the Kamioka signal time, IMB searched their data and found the signal, greatly strengthening the credibility of the two observations. The burst was seen also at Baksan Neutrino Observatory lying in the Caucasus Mountains in Russia. It had started operations in 1977, the first such observatory in the USSR. It consisted of the Baksan Underground Scintillation Telescope with the gallium-germanium neutrino telescope SAGE, the Soviet-American Gallium Experiment initiated in 1985 as a collaborative effort between the United States and the Soviet Union, designed to measure the flux of p-p neutrinos that are produced in the dominant energy-producing mechanism of the sun. The detection of even a few of the neutrinos from the supernova was epochal. In marking the beginning of extra-solar system neutrino astronomy, it confirmed our general understanding of stellar evolution in its final catastrophic stages. The observations of the neutrino burst not only unequivocally determined he nature of the supernova, but also fixed the time of core collapse, allowing identification of the progenitor star, a blue supergiant. This was an unexpected identification, because at the time a blue supergiant was not considered a possibility for a supernova event in existing models of high mass stellar evolution.
After this success, Koshiba's collaborators went back to their work on solar neutrino observation and after about half a year they could make the background subtraction and see the signal. But in the meantime, immediately after the supernova event, Koshiba had retired at the age of 60, and appointed Totsuka as spokesperson of the Kamiokande experiment, of course continuing to collaborate and act as a consultant, in particular for the preparation of the Super-Kamiokande proposal. He was invited to spend four months in Hamburg and one year at CERN. When he came back, he looked at the accumulated Kamiokande data and compared the fluxes for the two types of neutrinos, the ratio of muon neutrinos over electron neutrino fluxes and considered the problem of how those neutrinos were produced in the atmosphere. Primary cosmic rays striking the atmosphere produce pions and kaons, which subsequently decay into muons and muon-neutrinos and, much less abundantly, electrons and electron-neutrinos. The muons further decay into electron-neutrinos and muon-neutrinos (and electrons). As a consequence, it was expected that there were roughly two muon-neutrinos for each electron-neutrino and that the shape of the energy spectrum would be similar to that of the pions and muons. But the flux of muon neutrinos seemed considerably lower. There was a factor of 2, and Koshiba thought it was real. He checked the various causes and found that the same ratio could be derived from IMB data. He then wrote a draft, which circulated among the Kamioka collaborators, inviting objections and comments. In January 1988, the collaboration (27 people divided between Japan and the University of Pennsylvania) submitted an article on a detailed analysis of 277 events in the Kamiokande detector. They showed the existence of an apparent discrepancy between the observed ratio of atmospheric electron-neutrino-induced events over atmospheric muon-neutrino-induced events and the analogous number calculated by Monte Carlo numerical simulations of atmospheric decay processes. They had in fact developed a program, which simulated the atmospheric neutrino interactions in the detector using the atmospheric neutrino fluxes calculated for the Kamioka site. The number of muon-like events was found to be significantly less than the number expected from the atmospheric muon-neutrino flux, while the number of electron-like events was well explained by the expected electron-neutrino flux. They concluded that they were unable to explain the data as the result of systematic detector effects or uncertainties in the atmospheric neutrino fluxes, and added: “Some as-yet-unaccounted-for physics such as neutrino oscillations might explain the data.”
In 1989, Kamiokande-II published the results of their first observation in real time of the flux of boron-8 solar neutrinos using their imaging water Cherenkov detector, which yielded information on the neutrino arrival time, the direction, and the energy spectrum. The totality of the data provided a clear two-part evidence for a neutrino signal from the boron-8 production and decay in the Sun: namely, the directional correlation of the neutrino signal with the Sun and the consistency of the electron-energy distribution of the signal in shape and energy scale with that expected from boron-8 decay. Accordingly, the mechanism of energy generation in the Sun based on fusion reactions that give rise to boron-8 as a by-product appeared to be unequivocally confirmed by the detection of neutrinos which could only have originated in the core of the Sun. However, based on 450 days of data in the period January 1987 through May 1988, the measured flux was 0.46 of the value predicted by the solar model. Within experimental errors, the Kamiokande-II value was in agreement with the corresponding value obtained by Davis with his chlorine-37 radiochemical detector in essentially the same time period.
In the meantime, since the mid-1980s, the existence of the solar neutrino problem had become established, and over the following few years, the deficit of solar neutrinos was first confirmed by the Kamiokande experiment and then by experiments in Italy and Russia using massive detectors containing gallium, GALLEX and SAGE, and based on the neutrino-induced nuclear reaction which transmuted gallium-71 into germanium-71. These experiments provided for the first time information for the low energy part of the spectrum, being suitable to the detection of neutrinos emitted in the initial proton-proton chain reaction, which has an upper energy limit of 420 keV.
Kamiokande-II found no significant time variation of solar neutrino flux observations for the time period of January 1987 through April 1990. The conclusion from Kamiokande-II experiments was that it had identified solar neutrinos, because the electrons scattered by the incoming neutrinos recoiled predominantly in the direction of the Sun-Earth vector, that it had detected neutrinos deriving from boron-8 decay, thus confirming the nuclear fusion processes inside the Sun. However it had observed only about 50% of the expected boron-8 solar neutrinos, reconfirming the solar neutrino deficit.
Kamiokande-II was upgraded to Kamiokande-III and took data from December 1990. The results obtained by the Kamiokande-III were statistically consistent with the Kamiokande-II results, although the two experiments had different detector configurations and systematic uncertainties. The boron-8 combined neutrino flux from Kamiokande-II and Kamiokande-III was 49% to 64% of the standard solar models, as announced in 1996. The mystery of the missing neutrinos was continuing. Very few physicists had taken seriously the idea proposed by Gribov and Pontecorvo. Perhaps neutrinos behaved differently than physicists had assumed. The evidence favouring this possibility increased with time.
In late 1995, the tank of the new upgraded detector Super-Kamiokande began to be filled with water. It was 32 times larger than Kamiokande and 11200 photomultiplier tubes were ready to pick up the flashes of Cherenkov radiation emitted when one of the swarm of neutrinos collided with an electron or triggered a nuclear process spawning an electron. Super-Kamiokande was much larger than its predecessor, which originally held only 3000 tons, so that it was able to generate more data on solar and atmospheric neutrinos in a few months than the cumulative production of all detectors to that date. It started taking data in April 1996; in August 1998, the Super-Kamiokande Collaboration, which included Koshiba, published “Evidence for Oscillation of Atmospheric Neutrinos”, where they presented an analysis of atmospheric neutrino data from 535-day exposure of the new upgraded Super-Kamiokande detector: “Experimental biases and uncertainties in the prediction of neutrino fluxes and cross sections are unable to explain our observation. The data are consistent, however, with two-flavour muon-neutrino/tau-neutrino oscillations...”. The first results of the solar neutrino flux measurement from Super-Kamiokande obtained from data taken between May 1996 and June 1997 were presented in August 1998. The total flux of boron-8 solar neutrinos was consistent with the Kamiokande measurements and was 36% of the flux predicted by solar models.
Sufficient data had by that time accumulated and all solar neutrino observations reported significantly lower fluxes than the expectations of standard solar models, which on the other hand looked well established and reliable also according to recent measurements of the helioseismological vibrations seen on the solar surface. The difference between observations and predictions strongly suggested some neutrino properties beyond the standard model of elementary particles. These experimental observations supported the theory that the neutrino has non-zero mass, a possibility that theorists had speculated about for years, and that was related to the neutrinos’ capability to oscillate from one flavour to another. It thus seemed overwhelmingly likely that the source of the problem lay in the behaviour of the neutrino, and specifically in neutrino oscillations. However, there was no ``smoking gun'': it could be proven that there was a deficit of electron-neutrinos, but it could not be shown that they had transformed into some other type of neutrino.
Since 1984, Herb Chen had pointed out the advantages of using heavy water as a detector for solar neutrinos. Using heavy water would make the detector sensitive to two reactions, one sensitive to all neutrino flavours, which would allow a detector to measure neutrino oscillations directly. In the charged current interaction, a neutrino converts the neutron in a deuteron to 2 protons and an electron. In the neutral current reaction a neutrino dissociates a deuteron breaking it into its constituent neutron and proton. All three neutrino flavours are equally likely to participate in this interaction. Extremely low backgrounds were essential for these experiments. Heavy water had previously been suggested for neutrino detection, but availability and cost were a deterrent. Several kilotons of heavy water were required for the design of this detector that was assembled at about 2 kilometres underground in Sudbury, Ontario. SNO detector, using the unique properties of heavy water, where the hydrogen has an extra neutron in its nucleus, was able to measure both the flux of electron neutrinos by the charged current reaction and the total flux of neutrinos by the neutral current reaction.
In June 2001, a team of scientists from Canada, the United States and the United Kingdom announced the first scientific results of the Sudbury Neutrino Observatory (SNO), bringing “with a certainty of 99.9%”, the first clear and compelling evidence that neutrinos oscillate, transmuting into one another as they travel before reaching the Earth. This oscillation in turn implies that neutrinos have non-zero masses. Solar neutrinos were providing a means of studying the intrinsic properties of the neutrino themselves. The total flux of all neutrino flavours measured by SNO agreed well with the theoretical prediction of solar models, thereby solving a three-decade old problem and officially inaugurating the era of neutrino physics beyond the Standard Model.
In the meantime the Kamioka Liquid Scintillator Antineutrino detector KamLAND was built in the old KamiokaNDE cavity. The KamLAND detector started to collect data in January of 2002. It recorded anti-neutrino events stemming from particles emitted by the 51 nuclear reactors in Japan plus 18 reactors in South-Korea. KamLAND was at an average distance of about 175 kilometres from these sources and thus sensitive to the anti-neutrino oscillations. 515 days of data showed that without neutrino oscillations about 365 events were expected, while only 258 events were observed. KamLAND made the case for neutrino oscillations and mass “seemingly inescapable”.
That same December 2002, Raymond Davis and Masatoshi Koshiba were awarded the Nobel Prize in Physics “for pioneering contributions to astrophysics, in particular for the detection of cosmic neutrinos”. 1/2 of the Prize went to Riccardo Giacconi “for pioneering contributions to astrophysics, which have led to the discovery of cosmic X-ray sources”.
Bibliography
Bilenky S. M. (2012) Neutrino. History of a unique particle. The European Physical Journal H 38 (3): 345-404
Bilenky S. M. (2004) The history of neutrino oscillations. A report at the “Nobel Symposium on Neutrino physics”, Haga Slott, Enkoping, Sweden, August 19-24, 2004. http://arxiv.org/pdf/hep-ph/0410090v1.pdf
Koshiba M. (1992) Observational neutrino astrophysics. Physics Reports 220 (5-6): 229-381
Interview with Masatoshi Koshiba (1997) by David DeVorkin. American Institute of Physics. http://www.aip.org/history/ohilist/24870.html
Koshiba M. (2002) Birth of Neutrino Astrophysics. Nobel Lecture. http://www.nobelprize.org/nobel_prizes/physics/laureates/2002/koshiba-lecture.pdf
Bahcall J. and R. Davis Jr. (2000) The Evolution of Neutrino Astronomy. Publications of the Astronomical Society of the Pacific 112: 429-433