Prof. Dr. Mario José Molina > Research Profile
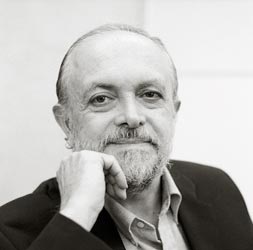
by Luisa Bonolis
Mario J. Molina
received the Nobel Prize in Chemistry 1995 together with Paul J. Crutzen and F. Sherwood Rowland "for their work in atmospheric chemistry, particularly concerning the formation and decomposition of ozone".
A passion for chemistry
Mario Molina was born in 1943 in Mexico City. He was already fascinated by science before entering high school, as he himself recalled in his autobiography: “I still remember my excitement when I first glanced at paramecia and amoebae through a rather primitive toy microscope. I then converted a bathroom, seldom used by the family, into a laboratory and spent hours playing with chemistry sets. With the help of an aunt, Esther Molina, who was a chemist, I continued with more challenging experiments along the lines of those carried out by freshman chemistry students in college.” Following the family tradition of sending children abroad for a couple of years, and aware of his interest in chemistry, his parents sent him to a boarding school in Switzerland when he was 11 years old, on the assumption that German was an important language for a prospective chemist to learn. At that time he had already decided to become a research chemist, even if earlier he had seriously contemplated the possibility of pursuing a career in music. In 1960, Molina enrolled in the chemical engineering program at the Universidad Nacional Autónoma de México (UNAM), as this was then the way to become a physical chemist, also taking math-oriented courses not available to chemistry majors.
After finishing his undergraduate studies in Mexico, Molina decided to obtain a Ph.D. degree in physical chemistry: “This was not an easy task; although my training in chemical engineering was good, it was weak in mathematics, physics, as well as in various areas of basic physical chemistry - subjects such as quantum mechanics were totally alien to me in those days. At first I went to Germany and enrolled at the University of Freiburg. After spending nearly two years doing research in kinetics of polymerizations, I realized that I wanted to have time to study various basic subjects in order to broaden my background and to explore other research areas. Thus, I decided to seek admission to a graduate program in the United States. While pondering my future plans, I spent several months in Paris, where I was able to study mathematics on my own and I also had a wonderful time discussing all sorts of topics, ranging from politics, philosophy, to the arts, etc., with many good friends.”
Subsequently, Molina returned to Mexico as an Assistant Professor at UNAM and he set up the first graduate program in chemical engineering. Finally, in 1968 he left for the University of California at Berkeley to pursue his graduate studies in physical chemistry. During his first year at Berkeley, he took courses in physics and mathematics, in addition to the required courses in physical chemistry. He then joined the research group of George Pimentel, with the goal of studying molecular dynamics using chemical lasers, which were discovered in his group a few years earlier. It was during this time that he had his first experience dealing with the impact of science and technology on society: “I remember that I was dismayed by the fact that high-power chemical lasers were being developed elsewhere as weapons; I wanted to be involved with research that was useful to society, but not for potentially harmful purposes.” After completing his Ph.D. degree in 1972, Molina stayed for another year at Berkeley to continue research on chemical dynamics. Then, in the fall of 1973, he joined the group of Sherwood Rowland as a postdoctoral fellow, moving to Irvine, California.
The atmospheric fate of the chlorofluorocarbon molecules
Rowland, who had pioneered research on “hot atom” chemistry, investigating chemical properties of atoms with excess translational energy produced by radioactive processes, offered Molina a list of research options: “The one project that intrigued me the most consisted of finding out the environmental fate of certain very inert industrial chemicals - the chlorofluorocarbons (CFCs) - which had been accumulating in the atmosphere and which at that time were thought to have no significant effects on the environment.” In the early 1970s, CFCs were a triumph of the chemical industry, already in wide and growing usage around the world. Invisible, nontoxic, nonflammable, and engineered to be chemically inert, these synthetic chemicals were known for their considerable stability and thus widely used as near-ideal coolants in refrigerators and air conditioners, in insulation and as propellants in many aerosol-spray products such as deodorants and hairsprays. CFC's do not readily undergo chemical reactions. Scientists at that time therefore believed that CFC's caused no harmful effects. Actually, one of the special advantages cited for this molecule was that it would be an excellent tracer for air mass movements because its chemical inertness would prevent its early removal from the atmosphere. Molina thus thought that this project offered him the opportunity to learn a new field - atmospheric chemistry - about which he knew very little; trying to solve a challenging problem appeared to be an excellent way to plunge into a new research area.
The CFCs are compounds similar to others that Rowland and Molina had investigated from the point of view of molecular dynamics; they were familiar with their chemical properties, but not with their atmospheric chemistry. Rowland had recently become interested in the atmospheric concentration of the man-made chlorofluorocarbon CCl3F during a Chemistry-Meteorology workshop organised by the Atomic Energy Agency. He had heard a presentation about recent measurements by the British scientist James Lovelock who had developed a highly sensitive device for measuring extremely low organic gas contents in the atmosphere, the electron capture detector. Using this, he could now demonstrate that the exclusively man-made, chemically inert, CFC gases had already spread globally and were accumulating throughout the Earth's atmosphere. Lovelock suggested tracking the CFC's to learn more about the atmosphere. He had developed a scientific instrument to measure CFC levels. Lovelock's shipboard observations showed the presence of CCl3F in both the northern and southern hemispheres, in quantities roughly comparable to the total amount manufactured up to that date.
The appearance in the atmosphere of a new, man-made molecule provided a scientific chemical challenge. Rowland had wondered: Was enough known about the physicochemical behaviour under atmospheric conditions of molecules such as CCl3F to allow prediction of its fate, once released into the environment? In early 1973, in submitting his regular yearly proposal to the AEC, which had sponsored his research involving radioactive tracer species since 1956, Rowland also included a predictive study of the atmospheric chemistry of CCl3F: what would eventually happen to the chlorofluorocarbon compounds in the atmosphere? Against this backdrop, in that same year, Mario Molina joined Rowland's research group as a postdoctoral research associate.
At first the research did not seem to be particularly exciting. Molina carried out a systematic search for processes that might destroy the CFCs in the lower atmosphere, but nothing appeared to affect them. Rowland and Molina knew that as synthetic molecules CFCs had been designed to be inert and nonsoluble and were unlikely to be decomposed by common atmospheric processes. But they also realised from laboratory data and chemical insight that the CFCs could not remain inert in the atmosphere forever. After CFC's are released into the atmosphere, they rise slowly and essentially nothing happens in the lower atmosphere, which can be reached only by the relatively low energy, long wavelength portion of the ultraviolet (UV) spectrum. However, it was their considerable stability, that allowed them to waft - one spray at a time - into the upper atmosphere where they would readily absorb highly energetic ultraviolet light (wavelengths below 220 nanometers) that usually causes the decomposition of simple atmospheric molecules. All multi-atom compounds are capable of absorbing UV radiation if the wavelength is short enough, and almost all will decompose after absorbing the radiation.
The search for any removal process that might affect CCl3F began with the reactions that normally affect molecules released to the atmosphere at the surface of the Earth. Several well-established tropospheric sinks – chemical or physical removal processes in the lower atmosphere - exist for most molecules released at ground level: Coloured species such as the green molecular chlorine, Cl2, absorb visible solar radiation, and break apart (photodissociate) into individual atoms as the consequence. Highly polar molecules, such as hydrogen chloride, HCl, dissolve in raindrops to form hydrochloric acid, and are removed when the drops actually fall; almost all compounds containing carbon-hydrogen bonds, for example CH3Cl, are oxidized in our oxygen-rich atmosphere. However, CCl3F and the other chlorofluorocarbons as CCl2F2 and CCl2FCClF2 are transparent to visible solar radiation and all radiation that penetrates to the lower atmosphere. They are basically insoluble in water and do not react with HO, O2, O3, or other oxidizing agents in the lower atmosphere. When all of these usual decomposition routes are closed, what happens to such survivor molecules?
CFC molecules in the lower atmosphere are protected against very energetic UV radiation by O2 and O3 molecules at higher altitudes. In the upper atmosphere, the UV wavelengths below 242 nm can be absorbed by O2, splitting it into two oxygen atoms (O2+UV light-->O+O), thus initiating a sequence of reactions. Both of these O atoms normally attach themselves to an O2 molecule to form ozone, O3. A collision with some third molecule, M, is needed to stabilise the O3 product (O+O2+M-->O3+M). These ozone molecules in turn can absorb ultraviolet radiation, with absorption increasing greatly in strength at shorter wavelengths in the UV, and split off an O atom (O3+UV light-->O+O2). Such absorption is especially strong for wavelengths shorter than 290 nanometres. Again, these O atoms usually reform ozone by reacting with O2. Ozone is actually quite chemically reactive and sometimes intercepts the O atoms forming two O2 molecules (O+O3-->O2+O2). This set of four reactions involving O, O2 and O3 was already recognised by Sydney Chapman, who formulated in 1930 the first photochemical theory for the formation and decomposition of ozone in the atmosphere. Through these two-step splitting-plus-combination and other processes a balance of ozone is maintained in the atmosphere by which about 3 parts in 107of the entire atmosphere are present as O3, versus almost 21% as O2.
About 90% of these ozone molecules are present at altitudes between 10 and 50 kilometres, i.e. in the stratosphere, where the mixing ratio of O3 can rise as high as 1 part in 105, while the troposphere contains only 10 % of the atmospheric ozone. The total amount of ozone in the stratosphere is nevertheless rather small; it corresponds to about the number of air molecules contained in a layer 3 mm thick at standard temperature and pressure. The solar UV energy absorbed by O3 is converted into heat, providing a heat source in the 30-50 km altitude range. This heat source creates the stratosphere, the region characterised by an inverted - that is, increasing - temperature profile that rises typically from 210 K at its base at 10-15 km altitude, to roughly 275 K at 50 km altitude.
Because both O2 and O3 can absorb short wavelength UV radiation, no solar radiation with wavelengths < 290 nm penetrates below the stratosphere. The ozone layer in the atmosphere thus performs two important physical processes: it removes the most energetic fraction of this radiation and changes UV energy into heat, maintaining the stratosphere. For nearly a billion years, ozone molecules in the atmosphere have protected life on Earth from the effects of ultraviolet rays. UV-B radiation (280- to 315- nanometer wavelength) from the Sun is partially absorbed in this layer. As a result, the amount of UV-B reaching Earth’s surface is greatly reduced, greatly diminishing risks of skin cancer, cataracts, suppressed immune system and possible damage to terrestrial plant life, single cell organisms, and aquatic ecosystems.
Detailed knowledge of the Earth's atmospheric ozone distribution seasonally and geographically was outlined beginning in the 1920s by a series of experimenters, especially G. M. B. Dobson, who began regular measurements with an UV spectrometer. Dobson realized that careful measurements of the relative intensities of solar UV radiation at different wavelengths could be converted into quantitative estimates of the amount of ozone overhead, and his initial work near Oxford established that the amount of ozone varied from day to day and month to month. The modern era of stratospheric ozone studies began in the 1950s with proposals that the detailed ozone source and sink equations might not be in balance. Rocket experiments were well underway, and extensive data were now available for the upper reaches of the atmosphere and for outer space. Also, the equipment for studies of chemical kinetics had improved greatly.
It was generally believed that the four reactions sufficed to explain the ozone concentration distribution in the stratosphere, but measurements now showed appreciable deviations from Chapman's theory. The calculated ozone contents were considerably higher than the observed ones. This led scientists to look for other chemical reactions contributing to the reduction of the ozone content. A conclusion began to be formed: less ozone, perhaps by a factor of two, was actually present in the atmosphere than would be expected if the Chapman reactions were the only significant contributors to its creation and removal. Because the formation reactions for ozone seemed solidly based, the “missing” factor was more likely that some additional removal process must exist for ozone. In the mid-1960s first proposals for the missing sink fell on a free radical catalytic chain initiated by HO in reactions absorbing O and O3 and releasing O2 (HO+O3-->HO2+O2; HO2+O-->HO+O2). In these reactions HO reacts catalytically, without itself being consumed. However, further measurements of the rates for these reactions showed that this proposal by itself was clearly insufficient. The discussion had, however, expanded the scientific thinking beyond the Chapman reactions, and had brought free radical reactions into the picture.
How did CFCs enter at this stage? The CCl3F molecule was known from laboratory studies to be able to absorb UV radiation at wavelengths < 220 nanometers, but to encounter such solar radiation in the atmosphere the molecule must first drift randomly through the atmosphere to altitudes higher than most of the O2 and O3 molecules - roughly to 25 or 30 km. More than 98% of the atmosphere and 80% of the ozone lies below 30 km altitude. In this rarefied air, the CFC molecules are exposed to very short wavelength UV radiation and decompose with the release of Cl atoms. Because at any given time, only a very small fraction of CFC molecules are found at altitudes of 30 km or higher, the average molecule survives for many decades before it is decomposed by solar UV radiation according to the reactions CCl3F+UV light-->Cl+CCl2F and CCl2F2+UV light-->Cl+CClF2.
In 1974 Rowland and Molina calculated the vertical profile to be expected for CCl3F in the stratosphere. The decomposition rate for this molecule escalates rapidly with increasing altitude in the 20-30 km range, so that the estimated average lifetime in the atmosphere was in the range from 40 to 55 years, while for CCl2F2 it was 75 to 150 years. However, once at the top of the atmosphere, the decomposition process is so rapid that a CFC molecule would last at most a few weeks if directly released there.
The answer to their original scientific question was that the eventual fate of the CFC molecules is photodissociation in the mid-stratosphere with the release of atomic chlorine, but on a time scale of many decades. The question was thus not only what destroyed CFCs, but more importantly, what the consequences were. What was the fate of these chlorine atoms at an altitude of 30 km?
The major chemical components of the mid-stratosphere were well known, and their reaction rate constants with atomic Cl had been measured in the laboratory. The newly released single chlorine atom, Cl, resulting from decomposition of CFCs, is chemically very reactive. During research promoted in the early 1970s by the Climate Impact Assessment Program, some researchers had turned their interest to the potential input of reactive chlorine radicals on stratospheric ozone. In the most thorough of these studies, Richard Stolarski and Ralph Cicerone calculated significant ozone depletions if inorganic chlorine were to be present in the stratosphere at a volume mixing ratio of 1 nanomole/mole of air. They were interested in the possible natural release of HCl to the atmosphere from volcanoes, or of man-made chlorine in the exhaust of solid rockets fuels scheduled for propulsion of the space shuttle. First, almost all chlorine atoms react with ozone by the reaction Cl+O3-->ClO+O2 forming another chemically reactive molecule ClO. The question then moved to the ClO product of the above reaction. What happened to ClO at 30 km? Atomic oxygen is especially abundant in the upper stratosphere between 30 and 50 km altitude. The ClO is also chemically reactive and reacts with atomic oxygen to re-release atomic chlorine (ClO+O-->Cl+O2), leaving a second molecule of diatomic oxygen. This reaction of ClO occurs in one or two minutes during the sunlit part of the day. The net result of these catalytic reactions is: O+O3--> 2 O2.
The study by Stolarski and Cicerone, first presented at a conference in Kyoto, Japan, in the fall of 1973 struggled, however, with the problem of a missing chlorine source in the stratosphere, because research had shown that the volcanic source is rather insignificant. But for Rowland and Molina the ClOx chain of reactions now became the key to understand the impact of the release of chlorine atoms from breakdown of CFCs in the stratosphere.
Since the radical Cl atom starts this whole reaction, but is not consumed in the process, it is a catalyst for the ozone destruction reaction. Within three months, they realized that when the catalytic efficiency of about 100,000 ozone molecules removed per chlorine atom is coupled with the yearly release to the atmosphere of about one million tons of CFC's, the original challenging and interesting scientific question had been converted into a potentially grave global environmental problem involving substantial depletion of the stratospheric ozone layer.
One evening, as that realisation was dawning, Rowland’s wife, Joan, asked how his research was going. “It’s going very well,” he said. “but it looks like, I think, the end of the world.” They were looking at an ozone removal process that was dominant over the normal processes in the stratosphere, changing the whole scientifically interesting problem into a serious environmental one.
They became fully aware of its seriousness when they compared the industrial amounts of CFCs to the amounts of nitrogen oxides, which control ozone levels. The role of these catalysts of natural origin had been established a few years earlier by Paul Crutzen. In 1970, Crutzen had showed that nitrogen oxides, present at parts per billion levels in the stratosphere, accelerate the rate of reduction of ozone by means of catalytic cycles. He had pointed out that emissions of nitrous oxide (N2O), a stable, long-lived gas produced by soil bacteria, from the Earth's surface could affect the amount of nitric oxide (NO) in the stratosphere. Crutzen showed that nitrous oxide lives long enough to reach the stratosphere, where it is converted into NO. In the reactions NO+O3-->NO2+O2 and NO2+O-->NO+O2, nitrogen oxides NO and NO2 react catalytically with ozone, thus accelerating the rate of reduction of the ozone content. Crutzen then noted that increasing use of fertilisers might have led to an increase in nitrous oxide emissions over the natural background, which would in turn result in an increase in the amount of NO in the stratosphere. Thus this kind of human activity, too, could have an impact on the stratospheric ozone layer. The connection demonstrated by Crutzen between microorganisms in the soil and the thickness of the ozone layer was one of the motives for the later rapid development of research on global biogeochemical cycles.
Rowland and Molina decided to exchange information with the atmospheric sciences community. They went to Berkeley to confer with Harold Johnston, who had carried out extensive laboratory studies of the chemistry of nitrogen compounds. In 1971, he had pointed out the possible threat to the ozone layer that the planned fleet of supersonic aircraft and supersonic travel might represent, in being capable of releasing nitrogen oxides right in the middle of the ozone layer at altitudes of 20 km. Crutzen's and Johnston's work gave rise to a very intensive debate.
Rowland and Molina publicised their results at a press conference in September 1974, soon after their first paper on the topic was published in the journal Nature. The two scientists showed that when CFCs rise into the stratosphere, they are bombarded by powerful doses of ultraviolet rays. A single chlorine atom knocked free, they found, can absorb more than 100,000 ozone molecules. More disturbingly, the atoms could linger in the stratosphere for up to a century.
This work was the first step in tracing the causal chain linking industrial production of CFCs with global ozone depletion demonstrating how certain human activities can have environmental impacts far beyond what one might intuit.
Their original calculations about the behaviour of the CFC species in the stratosphere were actually predictions of the vertical distribution because no measurements were then available for any chlorinated species in the stratosphere, and certainly not for the CFCs. During 1975, two different research groups sent evacuated containers equipped with pressure-sensitive valves up on high altitude balloons and recovered air samples from the stratosphere. The measured mixing ratios for CCl3F were in excellent agreement with the vertical profiles calculated by Rowland and Molina in the previous year. This fit between theory and experiment demonstrated both that CFCs reach the stratosphere and that they are decomposed there by solar ultraviolet radiation at the altitudes predicted.
In many public appearances, Rowland presented their case that continued use of CFCs would greatly diminish the ozone layer, thereby increasing the intensity of biologically damaging ultraviolet light at Earth's surface. Stratospheric chemistry at the time was based on balloon-borne samplers of trace gases and on one-dimensional models. Nonetheless, the pair measured the ultraviolet cross sections of CFC-11 and -12 in the lab, calculated their photolytic destruction rates in the atmosphere and derived their atmospheric lifetimes as 50–100 years. They reviewed industrial production and emission of CFCs, projected the build-up and release of chlorine atoms in the stratosphere and concluded that ozone depletion was likely and would be long-lived, even with remediation.
Founding atmospheric sciences
A great deal of media and public attention in CFCs ensued, especially between 1974 and 1978, including hearings in state legislatures, city councils, and the U.S. Congress. Industry representatives at first disputed Rowland and Molina's findings. By 1979, the United States and other nations had banned the sale of aerosol cans that contained CFCs, but companies continued to produce CFCs for other uses.
For a decade, Rowland and Molina persevered to prove their hypothesis, publishing numerous scientific papers and speaking to hostile audiences at scientific conferences. Cicerone, whose own work had established the possibility of a chlorine chain reaction, said: “He [Rowland] mentioned to me that he had not been invited to any chemistry department to give a lecture” from about 1975 to 1985. “The territory they stepped into and defined were so new that most scientists felt they didn’t know what was going on... They didn’t feel prepared - or they felt the linkage with an ongoing human activity was too big a step.”
But their findings, achieved in laboratory experiments, were supported 11 years later when British Antarctic Survey scientists Joseph Farman, Brian Gardiner, and Jonathan Shanklin detected recurring massive springtime losses of ozone over their survey station at Halley Bay, Antarctica. The station had been established in preparation for the International Geophysical Year of 1957-1958 and was equipped with a standard Dobson UV spectrometer for the measurement of total ozone. Since the late 1970s, observations showed that the average October ozone concentrations over Halley Bay had begun to decrease (spring in Antarctica corresponds to fall in the northern hemisphere). In 1984, values had dropped well below the values of the 1960s. It became clear that the loss of ozone began soon after the end of the polar winter darkness and proceeded very rapidly for the next several weeks into mid-October.
This totally unexpected phenomenon was reported in Nature in May 1985, together with the hypothesis that the ozone decrease was correlated with the increasing CFC concentrations in the atmosphere.
Further observations of substantially less ozone over Halley Bay in October in the 1980s were quickly shown to be characteristic of the entire South Polar Region by the total ozone mapping spectrometer instrument on the Nimbus-7 satellite. Measurements taken on other satellites over the entire sunlit Southern Hemisphere showed that the lowest October ozone values fell rapidly during the years. These data provided the scientific basis for the conclusion that the ozone losses over Antarctica develop during the special atmospheric conditions existing in the long cold months of polar darkness. High wind speeds cause a fast-rotating vortex of cold air, leading to extremely low temperatures. Unusual and heterogeneous chemical reactions occur on the surface of the frozen stratospheric cloud particles, driven by the heightened chlorine. About half the total column ozone is lost during austral spring. The spatial scale of Antarctic ozone loss is immense, covering nearly the entire continent and thus this phenomenon has been popularly christened the ozone ‘hole’.
Moreover, during the many decades of random diffusion through the lower atmosphere, these CFC molecules are able to intercept outgoing terrestrial infrared radiation and thereby contribute significantly to the Earth's warming by the greenhouse effect. Svante Arrhenius long ago recognised that the continued combustion of fossil fuels (coal, gas, oil) would result in higher concentrations of atmospheric CO2 and suggested that the incremental trapping of infrared radiation would eventually cause global warming. As part of the International Geophysical Year (I.G.Y.) of 1957-1958, CO2 monitoring stations were established and further measurements provided the experimental evidence that CO2 had been increasing in concentrations over the following decades. In 1975, Ramanethan recognised that the rapid growth in the concentration of CFC-12 and CFC-11 provided two important additional greenhouse gases, because - as with ozone, carbon dioxide and water vapour - they are capable of absorbing the infrared radiation emitted by the earth. The energy of this infrared radiation is not sufficient to destroy the CO2 or CFC molecules when absorbed and is merely converted into heat in the process.
In 1975, Molina had been appointed as a member of the faculty at the University of California, Irvine, continuing to collaborate with Rowland as assistant professor and conducting research on his own. He set up an independent program to investigate chemical and spectroscopic properties of compounds of atmospheric importance. After spending several years at Irvine, he decided to move to a non-academic position joining the Molecular Physics and Chemistry Section at the Jet Propulsion laboratory in 1982.
During the period 1976-86, Molina's research defined and refined the relevant kinetics of the compounds that act as “temporary reservoirs” for the free radicals responsible for catalytic ozone destruction. Around 1985, after becoming aware of the discovery of the seasonal depletion of ozone over Antarctica, Molina's research group at JPL investigated the peculiar chemistry which is promoted by polar stratospheric clouds, some of which consist of ice crystals. They were able to show that chlorine-activation reactions take place very efficiently in the presence of ice under polar stratospheric conditions; thus, they provided a laboratory simulation of the chemical effects of clouds over the Antarctic. Also, in order to understand the rapid catalytic gas phase reactions that were taking place over the South Pole, experiments were carried out in his group with chlorine peroxide, a new compound which had not been reported previously in the literature and which turned out to be important in providing the explanation for the rapid loss of ozone in the polar stratosphere.
Rowland and Molina’s scientific investigations, together with Paul Crutzen's researches and commitment, started an environmental movement that ended with a success story for global cooperation on a worldwide environmental threat. Regulations for the control of CFC emissions had first been introduced during the late 1970s for the use of CFC-11 and CFC-12 as propellant gases for aerosol sprays, but only in the United States, Canada, Sweden and Norway. Companies continued to produce CFCs for other uses. Moreover, there was no urgency by the international community to ban CFCs outright, and researchers were challenged to prove that the observed ozone depletion was well beyond natural variations. The 1985 discovery of the huge ozone hole above Antarctica answered this challenge and that same year a United Nations meeting in Vienna, Austria, had agreed to a convention concerning protection of the stratospheric ozone layer. After a series of rigorous meetings and negotiations, the Montreal Protocol on Substances that Deplete the Ozone Layer was finally agreed upon on 16 September 1987 at the Headquarters of the International Civil Aviation Organization in Montreal. A landmark international environmental treaty was signed by 70 countries agreeing to phase out the production and usage of CFCs and other ozone-depleting chemicals and to eliminate inventories of them. Today, nearly 200 countries are signatories, including the United States.
All this research work was instrumental in starting the field of atmospheric chemistry. The further manufacture of CFCs has been banned by the 1992 revisions of the 1987 Montreal Protocol of the United Nations. Atmospheric measurements have confirmed that the Protocol has been very successful in reducing further emissions of these molecules. Recovery of the stratosphere to the ozone conditions of the 1950s will occur slowly over the years because of the long lifetime of the precursor molecules. Nowadays, models suggest that the concentration of chlorine and other ozone-depleting substances in the stratosphere will not return to pre-1980 levels until the middle decades of this century. On the other hand, it appears that the increasing impact of greenhouse gas warming will delay or even prevent the recovery of ozone levels in some parts of the globe. The ozone layer over the tropics and mid-southern latitudes might not return to pre-1980s levels of ozone for more than a century, if ever.
In 1989, Molina returned to academic life, moving to the Massachusetts Institute of Technology, holding a joint appointment in the Department of Earth Atmospheric and Planetary Sciences and the Department of Chemistry. There he continued with research on global atmospheric chemistry issues including work at the interface of the atmosphere-biosphere, which is critical to understanding global climate-change processes. On July 1, 2004 Molina joined the Department of Chemistry and Biochemistry at University of
California, San Diego and the Center for Atmospheric Sciences at the Scripps Institution of Oceanography.
Rowland and Molina were jointly awarded the 1995 Nobel Prize for Chemistry with Paul Crutzen “for their work in atmospheric chemistry, particularly concerning the formation and decomposition of ozone”. It was the first time the Nobel Prize has recognised research into man-made impacts on the environment. The award culminated 20 years of study on the ozone layer by the researchers. In announcing the Prize the Royal Swedish Academy of Sciences said that the researchers had “contributed to our salvation from a global environmental problem that could have catastrophic consequences.” Had CFC emissions not been stopped, we now understand that in addition to their destruction of the ozone layer, their impact on climate (through their greenhouse effect) would be comparable to that of carbon dioxide.
These discoveries were about more than just stratospheric ozone, they were about the whole environment. The realisation that something done anywhere on the Earth could have effects somewhere else represented the start of the global era of the environment. In his autobiographical profile, Molina, who had become the first Mexican-born citizen to ever receive a Nobel Prize in Chemistry, commented: “When I first chose the project to investigate the fate of chlorofluorocarbons in the atmosphere, it was simply out of scientific curiosity. I did not consider at that time the environmental consequences of what Sherry and I had set out to study. I am heartened and humbled that I was able to do something that not only contributed to our understanding of atmospheric chemistry, but also had a profound impact on the global environment.”
Bibliography
Molina M. (1995) Autobiography. Nobelprize.org. http://www.nobelprize.org/nobel_prizes/chemistry/laureates/1995/molina-bio.html
Molina M. (1995) Polar Ozone Depletion. Nobelprize.org. http://www.nobelprize.org/nobel_prizes/chemistry/laureates/1995/molina-lecture.html
Rowland F. S. (2006) Stratospheric ozone depletion. Philosophical Transactions: Biological Sciences 361(1469) Reviews: 769-790