Prof. Dr. Isidor Isaac Rabi > Research Profile
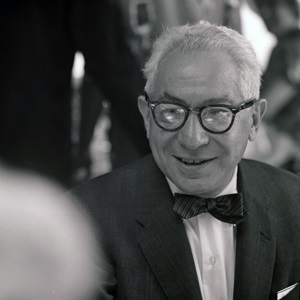
by Luisa Bonolis
Isidor Isaac Rabi
Nobel Prize in Physics 1944
"for his resonance method for recording the magnetic properties of atomic nuclei".
As Norman Ramsey, one of Isidor Rabi's biographers emphasised, "Some scientists make their greatest contribution through their own personal research, while others are best remembered for their general wisdom and their influence on others. A few, including Rabi, excel in both respects." It would actually be reductive to talk of Rabi's important discoveries, which led to his Nobel Prize in 1944, without mentioning how his influence extended far beyond his own laboratory and how, under his visionary leadership as a statesman of science, many successful ventures in national and international cooperation in science were realised. In particular, he was one of the founders of the Brookhaven National Laboratory and a main promoter of the CERN laboratory. His great reputation and his contacts, with the leading physicists as well as with the government leaders of the United Nations, turned into valuable tools when he became a spokesman for the peaceful use of nuclear energy.
Becoming an adept in quantum theory
Isidor Isaac Rabi was born in Rymanow, Austria-Hungary, in 1898, at the very end of the 19th century, when X-rays, radioactivity and the electron were discovered. The following year his parents moved to New York City where he attended public school, but gaining much of his education and interest in science through books borrowed from the public library. In 1916, after graduating from high school, Rabi entered Cornell University with a scholarship, starting in electrical engineering, but graduating in the field of chemistry. After three years far from university, he returned first to Cornell, to do graduate work in chemistry, moving a year later to Columbia University and turning to physics.
In 1923, when Rabi was beginning his physics studies, he discovered that his real interest was the quantum theory. However, no physics professor at Columbia was really conversant with such novelties coming from Europe and he had to choose a dissertation topic that involved measuring the magnetic susceptibility of a series of crystalline salts. In the meantime he organised a study group of fellow students to grapple with quantum mechanics. In July 1927, Rabi submitted his doctoral thesis to the journal Physical Review, and the next day he married Helen Newmark. Soon after, like many other US young physicists, he went on a travelling fellowship to Europe, in order to have a closer view of the pioneers of the new quantum mechanics.
A European Tour through the Centres of Quantum Mechanics
During the first months Rabi visited Erwin Schrödinger in Zurich, Arnold Sommerfeld in Munich, and Niels Bohr in Copenhagen. The latter arranged for Rabi to stay in Hamburg, with Wolfgang Pauli, who at the time was a collaborator of Otto Stern, one of the founding fathers of experimental atomic physics not including spectroscopy. In late October, Rabi arrived there with Yoshio Nishina, who was visiting Europe from Japan. Rabi well knew the Stern-Gerlach experiment of 1922, which had turned out to be one of the milestones on the path to modern quantum physics. In setting up this experiment, Stern was guided by Sommerfeld's extension of the Bohr theory of the atom – an extension independently put forward by Peter Debye – in which, in addition to the usual quantum numbers for the size and shape of orbits, a quantisation of the spatial orientation of the "Keplerian" electron orbits around the nucleus, was proposed, a proposal referred to as space quantisation. Because of the orbital motion of a single electron, an atom can possess a magnetic moment that determines its interaction with external electric and magnetic fields. Spatial quantisation allowed only selected discrete orientations of each atomic magnet relative to the direction of an externally applied magnetic field. In the Stern-Gerlach experiment a collimated beam of silver atoms, all with the same magnetic moment, flowing from a tiny hole of a heated furnace and moving with thermal velocities, passed through a strong non-uniform magnetic field. On its path between the furnace and the detector, the magnetic field will exert a torque on the magnetic dipole, which will thus precess about the direction of the magnetic field. The non-uniform field will also exert on the magnetic moment a transverse force, whose magnitude and direction depend on the orientation of the atom's magnetic moment relative to the direction of the externally applied magnetic field. The component of the magnetic moment parallel to the field direction will not be affected.
The classical picture includes no restriction on the angle at which the atomic magnet can precess about the magnetic field. The expectation is that, due to thermal effects in the oven, the magnetic dipole moments of the atoms will be randomly oriented in space with respect to the direction of the field. The directions of motion of the atoms in the initial beam would be displaced by random amounts perpendicular to the direction of motion of the initial beam. A continuous gradation of deflections should thus occur, and the transmitted beam would merely spread out like a fan.
In actual fact, Stern and Gerlach found that on the cold glass detector plate the parent beam split into two distinct parts -with no trace of silver atoms in the central region, where one would have expected the undeflected atoms- implying that, in the case of the silver atoms, only two distinct orientations are allowed with respect to the direction of the magnetic field. Stern and Gerlach thus considered their result a decisive refutation of the classical theory, disproving the classical Larmor theory, which was based on continuous values for the direction of the magnetic moments. But at the same time, they mistakenly considered the phenomenon a confirmation of the old quantum theory, according to which the magnetic moment of the silver atom was due to the electrons' orbital angular momentum.
Unknowingly, they had actually been the first to observe the quantisation of magnetic moment associated with electron spin, because their silver atoms were actually in the ground state, with total orbital magnetic moment equal to zero, so that the magnetic dipole moment of the atom was entirely due to the spin of the electron, a new quantum number that would be introduced in 1925 by George Uhlenbeck and Samuel Goudsmit.
The Stern-Gerlach experiment, an early triumph of the molecular beam method, offering other-than-spectroscopic evidence that quantum objects exhibit behaviour incompatible with classical physics, had stunned and intrigued Rabi as a student, when he was still sceptical about the quantum theory. He became convinced that the system of ideas underlying the Bohr atom and the attempts to extend these ideas to other atomic phenomena were well founded and began to study and discuss with his friends all the papers that would be gradually incorporated into the formal structure of the new quantum mechanics.
While working with Nishina and Pauli on theoretical work, he spent some time in Stern's laboratory and carried out successfully what became his first molecular-beam experiment. The magnetic field configuration he designed to deflect the beam particles became known as the Rabi field. Rabi's work in Stern's laboratory was decisive in turning his interest toward molecular beam research.
After Hamburg, Rabi went to Leipzig to work with Werner Heisenberg, but in the meantime Pauli left Hamburg for a chair in Zurich and in March 1929 Rabi and Robert Oppenheimer, whom he had met for the first time in Leipzig, followed him to Zurich. Once again it was a wonderful occasion of becoming acquainted with some of the finest minds in physics, but his stay in Zurich ended when, at the end of March, Rabi received a cable from Columbia University, offering him a lectureship at the physics department. They were searching for a theoretical physicist, who could teach the new quantum mechanics and Heisenberg himself, during a visit at Columbia, had strongly recommended Rabi for such a position. He accepted promptly, and on August 1, 1929, he left Europe with his young wife. His scientific apprenticeship had ended, he had developed a new awareness and knowledge of physics at the very sources of the new quantum mechanics.
Molecular Beams to Probe the Nucleus
Rabi devoted his first year at Columbia as lecturer exclusively to the strenuous effort of teaching the most advanced courses in the department. Thus began his pervasive influence on American physics. During the following two years he did theoretical research in solid-state physics, but his thoughts were very often directed to molecular beams.
In 1931, Harold Urey, Rabi's Columbia colleague, was attempting to determine the nuclear spin of sodium by an analysis of its spectrum, with inconclusive results. At the time, his longstanding involvement in isotope research was inspiring him to search for deuterium, the hydrogen-2 isotope, whose existence he actually announced in The Physical Review on New Year's Day, 1932. For this discovery Urey was then awarded the Nobel Prize in Chemistry 1934. Just seven weeks later, James Chadwick announced the ''possible existence of a neutron,'' a fundamental discovery which officially opened the nuclear era.
However, in 1931 the neutron was not yet there and the atomic nucleus was still a terra incognita, an unexplored territory soon to become the domain of Rabi's scientific adventure. Rabi saw that the molecular beam technique could be used to tackle the challenge offered by the uncertainty related to the nuclear spin of sodium. It could provide access to fundamental questions related to both the quantum world and the nuclear realm. Rabi wanted to measure the magnetic moment of a nucleus in the way that Stern had measured the magnetic moment of a silver atom. However, many refinements were required to transform the basic Stern-Gerlach experiment into a technique that could be used for quantitative measurements.
In principle, nuclear magnetic properties could be determined through the analysis of atomic spectra, but owing to the minute size of the nuclear moments – three orders of magnitude smaller than their electronic counterparts – experimental techniques were strained to the limit and it was quite difficult to get this kind of information via spectroscopy. Application of an experiment of the Stern-Gerlach type to the measurement of nuclear magnetic properties would provide an independent check on the difficult spectroscopic methods, and at the same time provide access to nuclear data that were otherwise unavailable.
With Gregory Breit, his colleague from New York University, Rabi had set up a joint seminar to explore and discuss atomic-nuclear phenomena. In 1931, they developed a formula that showed the variation of the magnetic moment of an atom for the different Zeeman levels of hyperfine structure under the influence of an external magnetic field. The beam method could thus be used to investigate the nuclear magnetic properties of atoms.
With Victor Cohen, his first graduate student, Rabi began his pioneering experimental work on the precise measurement of nuclear properties, which brought him to the forefront of nuclear physics during the following decade. By varying the deflecting fields along the path traversed by the sodium atoms, the beam was split into individual beamlets in each of which the sodium atoms were in the same hyperfine quantum state. The total number of beamlets depended on the nuclear spin of sodium, therefore, all they had to do, was count the number of beamlets observed at the detector. From this they could infer that the nuclear spin of sodium is 3/2, but for many months they did not communicate their findings, and the first experimental results were published only in March 1933.
In the same year 1933, Stern and his group had measured the magnetic moment of the proton, which was found to be about 2.8 times larger than what Paul Dirac's 1928 theory seemed to predict. This unexpected result was in fact a major discovery. The discovery of the spin of the electron had been of first importance in obtaining an understanding of atomic structure. Likewise, it was expected that a knowledge of the magnetic moment of the proton would play a similar role in the field of nuclear structure.
The fundamental character of the measurements of Stern and his collaborators prompted Rabi to set up his own experiment to measure the proton's - as well as the deuteron's - magnetic moment. With two postdoctoral fellows, J. M. B. Kellog and Jerrold R. Zacharias, Rabi quickly began to set up an experiment at Columbia University to measure the magnetic moment of the proton, by applying the Breit-Rabi theory.
Results published in 1934 indicated an even larger value than Stern's surprising result. Further attempts performed in 1936 utilised a new method with two deflecting magnets that each beam particle passed sequentially. After being deflected in the first inhomogeneous magnetic field, both fast and slow atoms would be refocused into the detector by the second inhomogeneous field, avoiding complications associated with the distributed velocities of the beam particles. Between the two deflecting magnets there was a new static, T-shaped field. Beams passing trough the static field saw the equivalent of a rotating, or oscillating, magnetic field, which exerted a tipping force on the magnetic moment making it flip from one orientation to another when the apparent field had an angular velocity approximately equal to the Larmor precession frequency of the magnetic moment about the magnetic field. The study of these stimulated transitions between magnetic states of the hydrogen atom allowed, for the first time, to determine that the magnetic moments of the proton and deuteron are positive. The effect of this new arrangement was that it greatly improved the experimental results, reducing the uncertainty in the measured value of the proton's magnetic moment from 10 percent to 5 percent and 4 percent instead of 26 percent for the deuteron. But not only did these results provide better values and the signs of the moment, but also the magnetic moment of the neutron.
The Magnetic Resonance Method
Throughout most of the 1930s Rabi and his collaborators, which by this time included also Polykarp Kusch, Sydney Millmann and Norman Ramsey, continued to investigate the first two isotopes of the hydrogen atom. In planning a third experiment, an apparatus very similar to that used in the preceding experiment was designed, but in a somewhat modified form. The two strong inhomogeneous deflecting fields were again set up to deflect beam particles in opposite directions, and the field strength of the second magnet was set to exactly undo what the first magnet did, that is to refocus the beam particles into the detector. If these two fields alone were acting on the beam, the number of atoms detected would be the same as if there were no fields present, because the second field would compensate exactly the action of the first field.
The real novelty of this experiment was that the third simple static T-field was supplemented by a weak field component superimposed at right angles to the strong constant homogeneous field and oscillating at an adjustable radio frequency. This oscillatory component could change the orientation of the precessing atoms inducing transitions (flipping over) of the magnetic moments just before they entered the second constant inhomogeneous field.
In full analogy to the resonance absorption of visible light, transitions to different quantum states could occur from one Zeeman hyperfine level to another if the alternating field satisfied Bohr’s frequency condition for the energy difference between the two levels. However, instead of optical frequencies one dealt here normally with frequencies in the radio range, because the differences between the energy levels are very small. Every molecule saw many cycles of the same frequency and the probability of a transition was thus enhanced. When the Larmor precession frequency in the static field matches the frequency of the oscillating field, many atoms flip to another orientation and miss the detector. In this case the detector registers a marked resonance minimum, the frequency position of this minimum being determined with the extraordinary precision achievable with the radio frequency gauge. When the Larmor frequency is no longer in resonance with the frequency of the oscillating field, the atoms are all refocused into the detector, and the signal is once again large.
This was the core of the magnetic-resonance method, the most significant improvement in molecular and atomic beam techniques, which clearly offered unprecedented accuracy in establishing radio relations with the world of the electron and of the atomic nucleus. Its most direct application was the measurement of nuclear magnetic moments. The basis for this is the resonance condition f=(μH)/Ih, in which f is the frequency of precession of the axis of nuclear spin in a magnetic field of strength H, and μ is the magnetic moment of the nucleus. The number I is the nuclear spin quantum number, an integer or half-integer, and h is Planck’s constant. The frequency of precession, once it is detected, is easily measured with high accuracy, and thus one can determine the quantity μ/Ih, and the magnetic moment can be found if the spin is known. Therefore, if the frequency of the oscillating field is slowly varied, a sharp decrease (the resonance phenomenon) occurs in the number of atoms arriving at the detector when the frequency of the field equals the Larmor frequency. Each such resonance then gives a value of the ratio μ/Ih and, hence, of the magnetic moment.
The first nuclear magnetic resonance curve was sent to Physical Review on January 15, 1938. Measurements on hydrogen with the resonance method continued in the late spring of 1938. As predicted, two strong resonances were observed with the molecule HD, one of which was associated with the proton, the other with the deuteron. Both these resonance absorptions made it possible to determine the magnetic moments of both the proton and the deuteron with improved precision. However, both the molecules H2 and D2 were presenting a pattern of different absorptions, instead of the single, strong narrow resonance the group expected. A new apparatus revealed the details of the multiple resonance pattern, but theory did not account for the obtained data, and Rabi soon realised that this might be due to the existence of another unsuspected property of the deuteron: a small but finite electrical quadrupole moment, which is a measure of lowest-order departure from a spherical charge distribution. This far-reaching discovery, announced in 1940, was quite a surprise. It immediately obliged theoreticians to renounce the central forces assumed to bind the neutron and proton together and to admit that nuclear forces are much more complex than the first nuclear models of the early 1930s had assumed.
After the gap in the annual succession of Nobel Prizes, due to the Second World War, it was not until the autumn of 1944 that the Royal Swedish Academy of Sciences announced that for 1943 the prize would be awarded to Otto Stern, "for his contribution to the development of the molecular ray method and his discovery of the magnetic moment of the proton", and that for 1944 to Isidor Rabi, "for his resonance method for recording the magnetic properties of atomic nuclei".
After World War II, nuclear magnetic resonance (NMR) became a workhorse for physical and chemical analysis. Still later, Rabi's discovery was extended to Magnetic Resonance Imaging (MRI), a powerful medical diagnostic tool, which is now used in medical centres the world over. In subsequent decades, the molecular beam method has been widely adopted by the physics and physical chemistry communities world wide, and about 20 Nobel Prizes were awarded for work based on the molecular beam method; among them there were Kusch and Ramsey, two of Rabi's former collaborators.
Bibliography
Krige, J. (2005) Isidor I. Rabi and CERN. Physics in Perspective 7: 150-164
Rabi, I. I., Interview by Thomas S. Kuhn, December 8, 1963. Niels Bohr Library \& Archives, American Institute of Physics, College Park, MD USA, http://www.aip.org/history/ohilist/4836.html
Rigden, J. S. (1983) Molecular Beam Experiments on the Hydrogens during the 1930s. Historical Studies in the Physical Sciences 13(2): 335-373
Rigden, J. S. (2008) Rabi, Isidor Isaac. In Complete Dictionary of Scientific Biography. Vol. 24. Detroit: Charles Scribner's Sons, Detroit. pp. 191-197. Gale Virtual Reference Library
http://go.galegroup.com/ps/i.do?id=GALE%7CCX2830906032&v=2.1&u=mpi_vb&it=r&p=GVRL&sw=w&asid=49bfc365b28fab40b3d973344d8135cf
Toennies, J.P. et al. (2011) Otto Stern (1888-1969): The founding father of experimental atomic physics. Annalen der Physik 523(12):1045-1070
Wasson T. (ed) (1987) Rabi, I. I. In Nobel Prize Winners, H. W. Wilson Company, New York, pp. 847-849