Prof. Dr. Sir George Paget Thomson > Research Profile
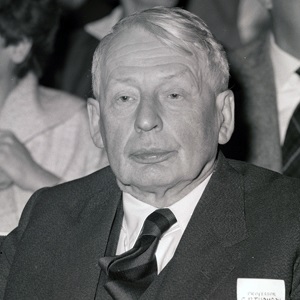
by Luisa Bonolis
George Paget Thomson
Nobel Prize in Physics 1937 together with Clinton Davisson
"for their experimental discovery of the diffraction of electrons by crystals".
George Paget Thomson was awarded the Nobel Prize for Physics in 1937 for his work in Aberdeen in discovering the wave-like properties of the electron. Whereas his father, J. J. Thomson, had been able to demonstrate the existence of the electron, the son, G. P. Thomson, demonstrated that it could be diffracted like a wave, a discovery proving the principle of wave-particle duality, which had first been posited by Louis-Victor de Broglie in the early 1920s. The Prize was shared with Clinton Joseph Davisson who had made the same discovery in the US, independently and following a quite different research path.
While Thomson maintained his interest in electron diffraction, in the early 1930s, after the discovery of the neutron and the positron and the related discovery of artificial radioactivity, he became deeply involved in the area of nuclear physics. In 1939, with the incredible expansion of the field following the announcement of the existence of the fission process, Thomson became instrumental in the establishment of the British wartime atomic energy project aiming at obtaining a controlled chain reaction. The dramatic events leading to the outbreak of World War II prompted Thomson to form the crucial MAUD committee of the Ministry of Aircraft Production in 1940--1941, which concluded that an atomic bomb was feasible. At the end of the war, Thomson became interested in the peaceful application of thermonuclear fusion and was instrumental in promoting the establishment of the British fusion programme as the first in the world.
Following his father's steps
George Paget Thomson was born in 1892 from a family of physicists, and bred in the privileged environment of the Cambridge elite. His father was Sir J. J. Thomson, Cavendish Professor of Experimental Physics from 1884 to 1919, director of Cavendish Laboratory, and subsequently master of Trinity College. His mother was Rose Paget, daughter of Cambridge Regius Professor of Medicine Sir George Paget, and herself a physics student of J. J.’s at the Cavendish Laboratory. On his mother's side he had a strong medical tradition, but both from his mother and father he derived a passion for mathematics and physics. In 1906, when G. P. was 14 years old, his father J. J. was awarded the Nobel Prize for Physics “in recognition of the great merits of his theoretical and experimental investigations on the conduction of electricity by gases.” His work showed that atoms are not the most fundamental units of matter, as had long been believed, opening the door to a new era in the physical sciences.
During his whole life, G. P. Thomson had a passion for model ship-building, that helped him develop his manual skill and apply his ingenuity. After attending King's College Choir School and Perse School, he won a scholarship to Trinity College, where he entered in October 1910. At Trinity he gained a double first in mathematics and physics and in 1911 he became a Major Scholar. After graduation, he started research in the Cavendish Laboratory under his father's supervision. At that time, J. J. was studying the characteristics of the so called “canal rays”, produced as positive rays in discharge tubes, separating the different kinds of atoms and atomic groupings present in them. During these experiments J. J. demonstrated that certain atomic groups, such as CH, CH2 and CH3, could be produced, even though they have no stable existence under ordinary conditions. He also found that samples of the inert gas neon contained atoms with two different atomic weights, an achievement in which he was greatly helped by his collaborator Francis Aston, who was working at the Cavendish Laboratory since 1910 on the invitation of J. J. Aston resumed this kind of experiments after the war constructing the first mass-spectrograph, a device that eventually provided the most satisfactory proof of the existence of isotopes among the elements in general. At the time, these studies added evidence to the existence of isotopes and proved important in the understanding of the heavy radioactive elements, such as uranium and radium.
J. J. Thomson's brilliant experimental researches and his excellent teaching attracted a host of the world's most talented young physicists to Cambridge University. Many of those who worked at the Cavendish under him eventually became Nobel laureates. His son G. P. was greatly influenced by this background to his early studies and experimental training. He also acknowledged the advantages he had at that time from interacting with Charles Wilson, who was in charge of the advanced teaching of practical physics at the Cavendish Laboratory until 1918. At that time, Wilson was perfecting his first cloud-chamber, which would become a fundamental tool for nuclear and particle physics in the years to come. Early in 1911, he was the first person to see and photograph the tracks of individual alpha- and beta-particles and electrons. But it was not until 1923 that the cloud chamber was brought to perfection and led to his two, beautifully illustrated, classic papers on the tracks of electrons. Rutherford's remark that the cloud chamber was "the most original and wonderful instrument in scientific history" has in fact been fully justified. Many important achievements were related to the use of the Wilson chamber. “For his method of making the paths of electrically charged particles visible by condensation of vapour,” Wilson was awarded the Nobel Prize in Physics in 1927. His technique was promptly adapted with startling success in all parts of the world, eventually leading in 1952 to the development by Donald A. Glaser of the bubble chamber, an achievement for which he was awarded the 1960 Nobel Prize in Physics.
In 1914, G. P. Thomson was elected Fellow and Mathematical Lecturer at Corpus Christi College, Cambridge, positions that he held until 1922 though he was absent from Cambridge on war service from 1914 to 1919. First, he served for a short time in France with the Queen's Regiment and then he was attached to the Royal Flying Corps for duty at the Royal Aircraft Factory at Farnborough and learned to fly at the Central Flying School. From the latter experience he derived an interest in aerodynamics that he applied to practical problems of stability and performance of aircraft, combining his skills as a mathematician, engineer and pilot, a combination of abilities that he used a lot in his later scientific life. After the war, he wrote Applied Aerodynamics, a comprehensive textbook on the subject outlining the basic physical theory and experimental methods applied to the aerodynamic performance of each part of an airplane's structure and to the airplane as a whole. His work on this field was of considerable practical value, giving to the subject a new degree of coherence and completeness.
When he returned to Cambridge in 1919, he resumed research on the behaviour of electrical discharges in gases, the work he had begun as a student under his father's direction. In the process he discovered, simultaneously with Francis Aston, that the element lithium occurs as two isotopes of masses 6 and 7. At that time, Aston had completed building his first mass spectrograph. Subsequent improvements of this instrument, employing electromagnetic focusing, allowed him to identify more than two hundred naturally occurring isotopes, with a much improved mass resolving power and mass accuracy. His work on isotopes also led to his formulation of the whole number rule which states that “the mass of the oxygen isotope being defined [as 16], all the other isotopes have masses that are very nearly whole numbers.” For his fundamental work, Aston was awarded the Nobel Prize in Chemistry in 1922.
Matter waves
In 1922, Thomson was appointed Professor of Natural Philosophy (Physics) in the University of Aberdeen, Scotland, a position he held until 1930 when he moved as professor of physics to Imperial College in London. It was at Aberdeen that Thomson made his most noteworthy contributions to theoretical physics. At first, he resumed his experimental work on a range of gas discharge phenomena, in particular those involving positive rays and he studied the field of forces existing within the hydrogen molecule between the protons and electrons. In 1925 he read with enthusiasm the recently published theory of matter waves of Louis de Broglie, bringing together the concepts of particle and wave in atomic physics.
In 1922, the young Louis, who worked in the spectroscopic laboratory of his brother Maurice in Paris, had suggested associating a small mass with a light quantum at rest, in order to treat it statistically like a material particle. He formulated the main ideas in three notes presented in September and October 1923 to the Paris Academy of Sciences. In Summer 1924 he completed the presentation of these concepts in a further note where one can also find the famous equation relating the wavelength lambda to the velocity of a non-relativistic particle (i.e., a matter particle and not the light quantum) of mass m, through Planck's constant h: lambda=h/mv. This was quite a revolutionary concept, since there was no evidence at the time that matter behaved like waves. In the early months of 1926, Erwin Schrödinger's remarkable series of papers on wave mechanics appeared, formalising de Broglie's ideas.
Thomson's interest in quantum theory and in de Broglie's ideas led him to work out a theory of the Bohr atom in which the constituent particles were described by a combination of this theory and his father's ideas on light-quanta as structures in the ether. He also reflected on problems related to the scattering of slow positive rays and slow electrons when, most probably towards 1925, he heard about studies on the interaction of electrons with metal surfaces performed by Clinton Davisson and his collaborators Lester Germer and Charles Kunsman, at Bell Laboratories in the US.
Beginning in 1920, Davisson and Germer had investigated the question: What is the nature of the secondary electron emission from grids and plates subjected to electron bombardment? For this project, Davisson was given a new assistant, Charles Kunsman, a new PhD from California. Soon after beginning their secondary electron emission studies, they observed an unexpected phenomenon: a small percentage (about 1%) of the incident electron beam was being scattered back toward the electron gun with virtually no loss of energy: the electrons were being scattered elastically. As he pictured it, the mechanism of scattering was similar to that of alpha ray scattering by a gold foil investigated in Geiger and Marsden experiments, following which Ernest Rutherford had announced his nuclear model of the atom in 1911. Davisson thus hoped to use these elastically scattered electrons to probe the extra-nuclear structure of the atom, just as alpha particles had been a probe for the nuclear core. He was so enthusiastic that he succeeded in obtaining permission to devote a large fraction of their time to this new project and had a specific apparatus built for this investigation. The basic piece was a vacuum tube with an electron gun, a nickel target inclined at an angle of 45° to the incident electron beam and a Faraday cup acting as collector, which could move through the entire 135° range of possible scattered electron paths. The Faraday cup was set at a voltage to accept electrons that were within 10% of the incident electron energy. After a couple of months they submitted a paper to Science, presenting their scattering program, the obtained curves and a shell model of the atom accounting for the observed angular distribution of electrons scattered by platinum and magnesium. But the data, the model, and the prediction were not definite, quite far from the Rutherford-Geiger-Marsden connection between experimental results and theoretical explanation. Notwithstanding the disappointment, Davisson and Kunsman continued these investigations for a couple of years, also building new tubes and trying other metals as targets, developing rather sophisticated experimental techniques at high vacuum. On the whole their scattering enterprise had a limited success, and, when Kunsman left Bell in the end of 1923, Davisson abandoned the project.
Investigations on the scattering of electrons by metals were resumed only in October 1924, when Lester Germer returned to work after a long period of illness. New experiments on a specially polished nickel target bombarded with electrons initially gave results very similar to those obtained during the previous years. Then suddenly, they obtained new unprecedented and puzzling results that appeared to have been caused by a change in the polycrystalline structure of the nickel target. The extreme heating had formed about ten crystal facets in the area from which the incident electron beam was scattered, and Davisson and Germer concluded that the new intensity pattern of the scattering electrons must have its origin in the new arrangement of the atoms in the crystals, not in the structure of the atoms. Neither Davisson nor Germer knew much about crystals, so they spent several months examining the damaged target and other nickel surfaces until they became thoroughly familiar with the X-ray diffraction patterns obtained from nickel crystals in various states of preparation and orientation.
At the time of Davisson and Germer's experiments, the study of crystals by X-ray diffraction was in full development, and its incredible impact on the chemical, biochemical, physical, material and mineralogical sciences was just beginning. The technique of X-ray diffraction had originated in 1912, when Max von Laue expressed the idea that the spacings of a crystal lattice would be of the right size to cause the diffraction of X-rays, supposing them to be very short electromagnetic waves. An experimental proof had immediately confirmed von Laue's hypothesis, and the technique had later been fully developed by Henry Bragg, and especially by his son Lawrence Bragg. They had both reduced X-rays analysis of simple materials to a standard procedure by 1914. Their findings created the new science of X-ray crystallography, a discipline later informing almost every branch of science, also leading to further developments in spectroscopy and solid-state physics and providing a means to understand the structure of highly complex molecules and materials, eventually giving rise to the field of molecular biology. Von Laue was awarded the 1914 Nobel Prize for Physics for his breakthrough discovery, while the Braggs, father and son, were jointly awarded the 1915 Prize for Physics “for their services in the analysis of crystal structure by means of X-rays.”
After spending several months in their investigation on crystals and on the examination of their damaged nickel target, Davisson and Germer decided that a large single crystal oriented in a known direction would make a more suitable target and by April 1926 they had obtained a suitable crystal and mounted it in a new set-up. However, after an entire year spent in preparation, they obtained quite uninteresting experimental results and Davisson was again highly disappointed. During the summer of 1926 he planned a vacation trip to visit England, which he had envisioned as a "second honeymoon" with his wife, but which would give him a completely new perspective of the whole matter.
Only a couple of years before Davisson and Germer's experiments, Arthur Compton had announced the outcome of five years of work, both experimental and theoretical, related to experiments on the scattering of monochromatic X-rays from electrons in a carbon target. According to what Compton had found, scattered X-rays had a longer wavelength than those incident upon the target, the shift increasing with the scattering angle. In 1923, he published a paper in the Physical Review where he explained his data by assuming that X-rays as electromagnetic quanta have a particulate nature and applied conservation of energy and momentum to the collision between the quantum of radiation and the electron. During the early 1920s, the particle nature of light suggested by Einstein in 1905 was still debated. Now Compton's experiments actually provided clear and independent evidence for the particle-like behaviour. Compton was awarded the 1927 Nobel Prize in Physics for the "discovery of the effect named after him."
At the time of Davisson and Germer's experiments, the dual wave-particle nature of light had thus just been proven. But at the same time, while defending his thesis on 25 November 1924, de Broglie had explicitly suggested a diffraction experiment on electrons by crystals in order to investigate his idea about the wave-particle duality of matter. In the meantime, several European physicists had attempted such experiments abandoning them because of the difficulties of producing the required high vacuum and detecting the low-intensity electron beams. In particular, in early 1925 Max Born's student in Gottingen, Walter Elsasser, became interested in the problem and suggested that some earlier experiments on slow electron scattering performed by Carl Ramsauer (the anomalous behaviour of the electron cross section for small velocities in noble gases), as well as the Davisson and Kunsman results, might be interpreted as evidence for the wave nature of electrons, the scattering patterns corresponding to those to be expected from wave diffraction. According to de Broglie's proposal, electrons have wavelengths that are inversely proportional to their momentum. Consequently, high-speed electrons have short wavelengths, comparable to the spacings between atomic layers in crystals. A beam of such high-speed electrons should undergo diffraction, a characteristic wave effect, when directed through thin sheets of material or when reflected from the faces of crystals. Elsasser checked the approximate magnitudes and found the electron wavelengths to be of the right order. He published a short note in Die Naturwissenschaften and also tried to perform an experiment to confirm his guess quantitatively, but did not succeed and eventually gave up.
De Broglie's papers, along with the new matrix mechanics of Werner Heisenberg, Max Born and Pascual Jordan, were the subjects of lively discussions at the Oxford meeting of the British Association for the Advancement of Sciences held in summer 1926. Davisson, who was largely unaware of these recent developments in quantum mechanics, attended the meeting. He was quite surprised in hearing a lecture by Born in which his and Kunsman's curves of 1923 were cited as confirmatory evidence for de Broglie's electron waves. Their feeble peaks actually appeared to be exciting evidence to physicists already convinced of the correctness of the wave mechanical interpretation of quantum theory.
After the meeting, Davisson discussed with Born, James Franck and Patrick Blackett, showing them some of the recent results that he and Germer had obtained with the single crystal. They convinced him that Elsasser's guess was correct and that his results were due to the effects of de Broglie waves.
Electron diffraction
After learning about matter waves and wave mechanics, Davisson spent the whole return transatlantic voyage trying to understand Schrodinger's papers, thinking that an explanation might reside in them. He had become convinced that, in view of the extremely fundamental nature of de Broglie's theory, it was highly desirable that it should rest on more direct evidence, and, in particular, that it should be shown capable of predicting as well as of merely explaining.
After his return, they examined the new curves that Germer had obtained during Davisson's absence and found a relevant discrepancy between the observed electron intensity peaks and the angles they expected from the de Broglie-Schrodinger theory. They carefully inspected the whole set-up and decided that most of the discrepancy could be accounted for by an accidental displacement of the collector-box opening. After a period of careful preparation, they started a new series of experiments to look for diffracted electron beams. Tests were specifically designed to see if any effect could be discerned for a changed electron wave-length, according to the de Broglie relationship. A search for “quantum peaks” (voltage-dependent scattered electron beams) was launched by late December, together with a new assistant, Chester Calbick, a recently graduated electrical engineer.
In January 1927, they found conclusive evidence of an interference phenomenon resulting from electron diffraction by a single crystal of nickel. They had actually succeeded where outstanding experimenters like Elsasser, Blackett, Chadwick and Ellis, had failed, even if they had in mind the idea of electron diffraction considerably ahead of Davisson and Germer. Recalling his conversations at Oxford, and the comments that had been made, Davisson felt urged to publish, feeling that others might be pursuing similar investigations.
Actually, unknown to Davisson, G. P. Thomson at that time was indeed making progress at revealing the phenomena of electron diffraction with high-voltage electrons and thin metal foils. He had learned of Davisson's studies in September 1926, while they were both attending the British Association Oxford meeting. Already deeply interested in de Broglie's work, Thomson immediately saw how his work with positive rays might be adapted to test the idea. In his case, the path from theory to experiment was linear, in contrast to Davisson and Germer, who arrived at their discovery in the course of a long lasting investigation that can be fully understood only within the research and development activities at Bell Laboratories. G. P. Thomson worked in a small university, whereas Davisson and Germer worked in a large industrial laboratory in the midst of a great city. The technical virtuosity of the low-energy experiments of Davisson and Germer, conducted as part of a more general investigation, is in strong contrast to the more directly targeted high-energy transmission experiments of Thomson and Reid.
According to the theory, a wave must be associated with the motion of any material corpuscle; a direct test could be obtained by producing a diffraction phenomenon when a beam of electrons went through a crystal lattice, the same test that Max von Laue and his associates had used in Munich to prove the wave nature of X-rays in 1911. Reasoning that the effect should be easier to analyse with a solid than with a gaseous target, Thomson asked one of his students, Alexander Reid, to modify an existing apparatus and investigate the scattering of a beam of electrons with energy in the keV range though thin celluloid films at normal incidence. Many of the energetic electrons that passed through the film were deflected to form diffuse rings on a photographic plate behind the target, quite similar to the Debye-Scherrer rings, well known from the corresponding experiment with X-rays. An interference phenomenon was at once suggested. This would occur if each atom of the film scattered in phase a wavelet from the incoming wave associated with the electrons forming the cathode rays. Since the atoms in each small crystal of the metal are regularly spaced, the phases of the wavelets scattered in any fixed direction would have a definite relationship to one another. In some directions they would agree in phase and build up a strong scattered wave, in others they would destroy one another by interference. The strong waves are analogous to the beams of light diffracted by an optical grating. At the time, the arrangement of the atoms in celluloid was not known with certainty and only general conclusions could be drawn, but for metals the structure had been determined previously by the use of X-rays. So Thomson and Reid changed to metal targets (aluminium, gold, platinum) with a known crystal lattice. In each instance, the deflected beam formed well-defined rings of a size that was in excellent agreement (to within 5 percent) with predictions from de Broglie's wave theory of matter. Thomson and Reid made a remarkable modification of the experiment that is worth mentioning. The beam, after passing through the film, was subjected to a uniform magnetic field. It was found that the beam whose impact on the plate formed the ring pattern was deflected in the same manner as the beam that had passed through holes in the film. This distinguished the effect from anything produced by X-rays and showed that it must be a true property of electrons. The experiments beautifully confirmed the de Broglie-Schrödinger wave theory.
These results were published in brief in December 1927 in Nature. Two months later, Thomson wrote two more detailed articles in the Proceedings of the Royal Society. The conclusion was: "The detailed agreement shown in these experiments with the de Broglie theory must, I think, be regarded as strong evidence in its favour. These means accepting the view that ordinary Newtonian mechanics (including the relativity modifications) are only a first approximation to the truth, bearing the same relation to the complete theory that geometrical optics does to the wave theory. However difficult it may seem to accept such a sweeping generalisation, it seems impossible to explain the results obtained except by the assumption of some kind of diffraction, and the numerical agreement with the wave-length given by the theory is striking."
Meanwhile, Davisson had published in April 1927 his own results on the scattering of slow electrons by single crystals in terms of de Broglie's theory. Both results established the wave nature of the electron, and, in principle, of all matter. The simultaneous discovery of electron diffraction is characterised by great differences in the two experimental techniques, in the institutional contexts, and in the scientific paths that eventually led to the final achievement. In his Nobel Lecture, Thomson himself testified to the magnitude of Davisson and Germer's technical achievement as a triumph of experimental skill, mainly because the relatively slow electrons they used were most difficult to handle and the vacuum had to be quite outstanding, in order to get results of any value: "If one compares the two sets of experiments it is clear that those of Davisson and Germer were great triumphs of experimentation, among the greatest ever made. Those at Aberdeen were singularly simple and easy, the only serious difficulty being to prepare good specimens. It is not hard to see the reason for this difference. Davisson had discovered an effect that he thought might be important in a particular way. He had to study this effect more or less as he had found it."
During the fifth Solvay Conference held in Brussels in October 1927, Bohr, de Broglie, Born, Heisenberg and Schrödinger all hailed the experiments of Davisson and Germer as confirming the general provisions and even the formulas of wave mechanics. The probabilistic interpretation of the wave-function, proposed by the physicists of the so-called Göttingen-Copenhagen school, provided the general conceptual framework in which the wave-corpuscle dualism found a theoretical coherence, and two years later the 1929 Nobel Prize in Physics was awarded to Louis de Broglie "for his discovery of the wave nature of electrons".
Davisson and Thomson were awarded the Nobel Prize in 1937, “for their experimental discovery of the diffraction of electrons by crystals.” Illness prevented Thomson from attending the award ceremony, but he travelled to Stockholm the following year to deliver his Nobel lecture. After some initial thanks and comments, he opened his talk with the following sentence: "The goddess of learning is fabled to have sprung full-grown from the brain of Zeus, but it is seldom that a scientific conception is born in its final form, or owns a single parent. More often it is the product of a series of minds, each in turn modifying the ideas of those that came before, and providing material for those that come after. The electron is no exception." In outlining the history of the electron, he recalled how during the last years of the XIXth century, the electron, as "atom of electricity", had "acquired not only mass but universality, it was not only electricity but an essential part of all matter." Among the many names associated with this advance, Thomson could not avoid mentioning his father J. J. who had been able to demonstrate the existence of the electron, whereas he, the son, had demonstrated that it could be diffracted like a wave.
The path was now open to study crystal structures by electron diffraction. X-ray diffraction by crystals is a powerful tool to probe the depths of metals and other crystals. Electrons, however, interact more strongly with matter than do X-rays and hence can be used for more sensitive studies of small samples, such as gas molecules, thin films, or surfaces. X-rays are useless for such surface and thin film studies. They are so piercing that they go right through the sample without disclosing the desired information. Electrons - far less piercing - are stopped, scattered and reflected by crystal surfaces and thin films and thus are vitally useful for this type of research.
A long period elapsed before improvements in electron optics and in vacuum technology enabled the unique properties of electrons to be widely used as a sensitive probe of the local microstructure and composition of matter. Electron diffraction, as well as neutron diffraction, discovered by von Halban and Preiswerk in 1936, became an extremely important tool, providing information on the structure of matter - crystalline and non-crystalline - at the atomic and molecular levels. Electron microscopy and diffraction-contrast methods developed and supplanted X-ray diffraction as the dominant microstructural tool in many laboratories, in materials science, chemistry, mineralogy, and biology.
An indication of the importance and diversity of applications of diffraction by crystals is given by the large number of Nobel Prizes awarded for studies involving X-ray, neutron, or electron diffraction.
Bibliography
Gehrenbeck R. K. (1978) Electron diffraction: fifty years ago. Physics Today 31: 34--41
Heilbron J. L. (2008) Thomson, Joseph John. in Complete Dictionary of Scientific Biography. Vol. 13. Detroit: Charles Scribner's Sons, pp. 362-372. Gale Virtual Reference Library,
http://go.galegroup.com/ps/i.do?id=GALE%7CCX2830904305&v=2.1&u=mpi_vb&it=r&p=GVRL&sw=w&asid=a4b35784121009d2caa74c2d733d8e67
Heilbron J. L. (1963) Interview with Sir George Paget Thomson June 20;
http://www.aip.org/history/ohilist/4913.html
Moon P. B. (1977) George Paget Thomson. 3 May 1892 -- 10 September 1975. Biographical Memoirs of Fellows of the Royal Society 23: 529-556
Thomson G. P. (1938) Electronic Waves. Nobel Lecture (June 7);
http://www.nobelprize.org/nobel_prizes/physics/laureates/1937/thomson-lecture.html
Wasson, T. (ed.) (1987) Thomson, G. P. In Nobel Prize Winners, H. W. Wilson Company, New York, pp. 1053--1054