Prof. Dr. Cecil Frank Powell > Research Profile
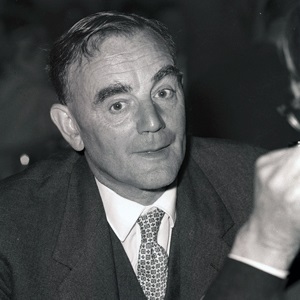
by Luisa Bonolis
Cecil Frank Powell
Nobel Prize for Physics 1950 "for his development of the photographic method of studying nuclear processes and his discoveries regarding mesons made with this method".
Early encounter with the cloud chamber
Cecil Powell was born in 1903 at Tonbridge, Kent. When he was a boy, his grandfather used to give him textbooks on the sciences. A book on chemistry especially excited his imagination and aroused his desire to make for himself some of the things described therein. In particular, he wanted to generate the gas hydrogen by the action of dilute sulphuric acid on granulated zinc. He saved money from his birthday and Christmas gifts and was able to buy materials and apparatuses to perform his experiment, which resulted in an explosion. Powell was luckily unhurt, but all the apparatus and glassware were completely destroyed. At school, he was an excellent student and was fortunate to come under the influence of a physics master who was very devoted to his profession and an able teacher, building circuits and designing original experiments with great skill. All this attracted Powell's attention toward physics. He won a scholarship to Sidney Sussex College, Cambridge, where he graduated with first-class honours in natural science in 1925. At first, he decided to follow his grandfather and to become a teacher. He was offered a teaching position at Uppingham School, but he soon became more and more uneasy about his suitability for a career of that kind. He chose to remain at Cambridge for graduate studies and asked Rutherford, - who had succeeded JJ Thomson as Cavendish professor of Physics at Cambridge in 1919 - if he could be accepted as a research student. Rutherford agreed and arranged for him to work with C. T. R. Wilson as his supervisor.
Wilson had achieved great fame for his invention of the cloud chamber, which allowed the tracks of ionising particles passing through a gas to be made visible: a cylindrical, glass-walled chamber is provided with a piston whose sudden motion causes the enclosed gas to expand. The gas, often ordinary air, is initially moist with water vapour, and the sudden expansion causes a sharp drop in its temperature so that the air can no longer hold the original amount of vapour in it; it becomes 'supersaturated' and some of it must condense. If at the moment of expansion, charged particles, such as alpha-particles from radioactive sources, are admitted to the chamber, the excess water condenses upon the charges or ions along the track. The trajectory of the particles then shows up as lines of droplets forming on the ions liberated by the passage of the fast particle through the atoms of the air. The tracks of the particles are recorded by taking flash photographs immediately following the expansion. The Wilson cloud method allowed atomic phenomena to be demonstrated in a most vivid way and showed the essential correctness of the picture of nuclear processes, which had been built up on the basis of a mass of indirect evidence. It also provided the first of a number of devices in nuclear physics that allowed far-reaching conclusions to be reached, with some confidence, on the basis of a single observation.
At that time Wilson had just brought to perfection this device, which Rutherford himself considered “the most original and wonderful instrument in scientific history.” This extraordinary tool, that was soon promptly adopted with startling success in all parts of the world, would soon become fundamental for nuclear and particle physics. Many important achievements and discoveries were obtained with the cloud chamber, fully justifying Rutherford's remark. For his invention Wilson was awarded in 1927 the Nobel Prize for Physics.
In 1925, when Powell arrived at Cavendish from Manchester, Rutherford had already discovered that by bombarding nitrogen with fast alpha particles from radium “atoms of hydrogen” were ejected - Rutherford named them protons - with the concomitant production of a new heavier nucleus, which turned out to be a rare oxygen isotope. The discovery of the first observed nuclear reaction in 1919 was made by counting the scintillations produced by the ejected protons on a zinc sulphide screen observed through a microscope. This work involved long periods in darkness and was slow and limited in its effectiveness. At the Cavendish, Rutherford continued these experiments on the transmutation of the lighter elements in close collaboration with James Chadwick using the scintillation method to find out what happened when fast alpha particles hit the nuclei of various light elements. But he was very impatient to have the cloud chamber perfected for the study of alpha particles bombarding targets and some time before had already asked Patrick Blackett to improve it. Blackett had been able to obtain very sudden expansions and to repeat them as frequently as desired. In 1924, work with the new chamber had started in earnest and Blackett took 23,000 photographs within a few months. After examining about 400,000 tracks, eight forked tracks were found which had a quite different appearance from those in a normal elastic collision. They were readily identified as the sought for transmutation of nitrogen. Photographs showed the capture of an incident alpha particle by a nitrogen nucleus, creating an isotope of oxygen, and the path of a very fast proton ejected from the recoiling oxygen nucleus. This beautiful result provided unambiguous and visible evidence both for the disintegration and for the synthesis of a heavier atom from a lighter, fully confirming Rutherford's expectation that one element can be artificially transmuted into another.
With the idea of further improving the cloud chamber, Powell built an all-glass device to study how the condensation of water droplets in dust-free air was affected by the degree of expansion of the chamber. The original intention was to determine whether better track photographs could be obtained by operating the chamber at other than room temperature. Powell concluded that there was nothing to be gained in this sense, although the results did help him to show that supersaturation in rapidly expanding steam accounted for an anomalously high rate of discharge of steam through nozzles. This discovery had significant consequence for engineering, contributing to the design of steam turbines.
Entering the field of nuclear physics
In the spring of 1928, Powell moved to Bristol as research assistant to A. M. Tyndall, who was soon greatly impressed by Powell's experimental ingenuity and skills. Powell's work during the first years was concerned with measuring the mobilities of positive ions in gases. However, in the early 1930s nuclear physics suddenly became a field in rapid expansion. A new era was inaugurated by Chadwick's demonstration of the existence of the neutron in 1932, an achievement for which he would be awarded the Nobel Prize for Physics in 1935. Between 1932 and 1933, physicists all over the world were excited by the discovery of the positron made by Carl Anderson at Caltech and of the pair production of positive and negative electrons by Patrick Blackett and Giuseppe Occhialini, who had married the cloud chamber technique with the electronic coincidence circuit developed by Bruno Rossi. Blackett and Occhialini had placed one Geiger-Müller counter above the chamber and another below, arranged in a coincidence circuit so that only particles passing through both counters, and therefore lying in the plane of the chamber, triggered the expansion. The particles were taking their own photographs. The result was that Blackett and Occhialini had multiple tracks on all plates. They explicitly linked the observed pair formation to Dirac’s theory of the relativistic electron placing it into a theoretical perspective. Antimatter had officially entered the realm of physics. Soon after, a surprising event opened the path to a new series of developments. The discovery of artificial radioactivity, announced in January 1934 by the couple Irène Curie and Frédéric Joliot, stimulated Enrico Fermi to successfully use the neutron, instead of charged alpha particles, as a projectile for bombarding the nucleus. The subsequent discovery of the effectiveness of neutrons slowed down in hydrogenated substances to induce radioactivity, opened the way to the study of nuclear reactions and to a new series of investigations on the structure of the nucleus. Both the Joliot-Curies and Fermi were eventually awarded the Nobel Prize in Physics for these important achievements.
However, when the actual discovery of the neutron was still in the future, the greater part of information on the structure of the nucleus had come from experiments with charged particles, alpha particles coming from radioactive sources. This procedure was not too promising for the large-scale investigations that Rutherford wanted to perform at the Cavendish Laboratory. The need to have more powerful beams led to the development of what became the Cockcroft-Walton accelerator that in early 1932 produced the first artificial nuclear reaction. Lithium-7 bombarded with protons occasionally captured a proton and the resulting nucleus of mass 8 disintegrated into two alpha particles with a total energy release of several million electronvolts, also providing a demonstration of Einstein's prediction on the equivalence of mass and energy. The success of this experiment prompted several laboratories to build their own accelerator.
As a result of these decisive discoveries, Powell became very interested in nuclear physics. In 1935, discussions on the necessity of investigating some branch of nuclear physics in Bristol resulted in Powell assuming the responsibility for constructing a 750 kV Cockcroft-Walton machine, and, in due course, a cloud chamber to register tracks from its highly focused proton beam. Entering the realm of nuclear physics completely changed Powell's research path. The original intention was to study the scattering of fast neutrons by protons using a cloud chamber filled with hydrogen, the neutrons being generated in the disintegration of light elements, such as lithium, beryllium and boron by the fast deuterons from the Cockcroft-Walton. But around this time something happened that led Powell into the exciting new field of subnuclear reactions generated by high energy cosmic-ray particles.
A new visual method: nuclear emulsions
It was between the end of 1937 and the beginning of 1938 that Powell found a scientific subject that became peculiarly his own. At that time, Walther Heitler, in Bristol from 1933 to 1941, showed Tyndall a publication by the Austrian physicists Marietta Blau and Hertha Wambacher, who had successfully used a photographic emulsion exposed to cosmic rays in the Austrian Alps. They had observed the tracks of low-energy protons as well as “stars”, nuclear disintegrations that had presumably been produced by cosmic rays. A photographic emulsion consists of myriads of small crystalline grains of silver bromide, with a small admixture of iodide, suspended in gelatine. The emulsion is commonly coated as a thin layer on to glass or celluloid. In normal photography, when light falls on the emulsion it produces minute changes in some of the grains so that when the plate or film is immersed in a chemically reducing solution, called the developer, some of the grains affected by light are turned into black grains of silver whilst the others are unchanged. If subsequently the plate is immersed in a “fixing” solution, the unaffected grains are dissolved out of the gelatine whilst the silver grains remain. The plate is then washed in water to remove the chemicals introduced into the gelatine by the fixing and other solutions; and the plate is subsequently dried to produce the finished photographic negative. The place where no light fell is now transparent, and blackest where the highlights fell. The action of fast charged particles is similar to that of light. The moving particle produces changes in some of the grains through which it passes so that, after processing, the track shows up as a line of developed grains, which can be seen and recognised when the plate is examined under the microscope at high magnification.
Powell was impressed by the relative simplicity of the method. They exposed an ordinary photographic plate on the laboratory roof, and on examining it with a microscope they were able to see some tracks and a “disintegration star”, that is the patterns of particle tracks from nuclear reactions of cosmic-ray particles with nuclei of the photographic emulsion.
Heitler suggested that they might begin by sending similar plates on to a mountain to see if they could simulate the Viennese results. In the experiments on the cosmic radiation it was only necessary to place a number of small plates coated with a suitable emulsion at high altitudes on a mountain where radiation is more intense than at sea level. To keep out light, the plates are wrapped in black paper, which the cosmic rays readily penetrate, and they are recovered after a few weeks, brought home to the laboratory, and processed. Following Heitler's suggestion to perform absorption experiments in air and lead, Powell devised the box of half-tone photographic plates - which were normally used for making lantern slides - and lead sheets that Heitler carried to the Jungfraujoch that same summer 1938.
They found “stars”, points from which the tracks of several charged particles diverged, which certainly represented disintegrations of nuclei from which several, sometimes as many as ten, charged particles, protons and alpha-particles had been ejected. They were certainly due to the collision between one of the silver or bromine nuclei in the emulsion and a fast proton or neutron, part of the cosmic radiation, which had an energy many times greater than those which were available with the most powerful accelerators in operation at that time. Encouraged by these results, Powell himself considered the possibility of using photographic emulsions in their experiments with the 750 kV accelerator to detect the neutrons and their scattering in place of the hydrogen-filled cloud chamber, as first proposed. He exposed a plate tangentially to his proton beam. Impressed by the results he then thought of using the technique with knock-on protons produced in hydrogenous materials by collisions with fast neutrons, in order to determine the energy spectra of neutron sources.
By measuring the ranges of these recoiling protons, their distribution in energy and thence that of the neutrons from which they have recoiled can be inferred. At the time, this method was the basis for determining the distribution of energy of the neutrons emitted in the disintegration of light elements, the neutrons being detected in a cloud chamber filled with hydrogen. But the method was arduous and in a characteristic experiment 30,000 photographs had to be taken to establish a particular neutron spectrum so that the whole experiment might take six months. When using the Cockcroft-Walton generator, they placed a few square centimetres of a half-tone emulsion near a “target” of beryllium bombarded with 700 kV deuterons. They recorded thousands of recoiling protons and were able to determine the neutron 'spectrum' with a higher precision than had been obtained with the cloud chamber, the measurements being completed in one or two days only.
This work inaugurated Powell's research in devising precise ways of measuring track characteristics and to develop the photographic emulsion method into a precision tool for nuclear and particle physics. Ignoring the view of the experts of the time, who claimed that the photographic method was not suitable for quantitative work, he became convinced that with precision microscopes, proper attention to processing, and emulsions of greater sensitivity and thickness the technique would prove to be of great value. In late 1939, Chadwick, who was already involved in fission studies at Liverpool University, learnt of Powell's emulsion technique for neutron detection and invited him to use the new 4 MeV cyclotron to extend his scattering experiments. From 1940 onwards, Powell applied the method to high-energy neutron spectroscopy as part of the British Atomic Energy Project.
Nuclear emulsions and cosmic ray studies
During the war years, Powell also kept an eye on the plates exposed on the Jungfraujoch immediately before the war and the possibilities of the cosmic-ray work. Between 1936 and 1938, Anderson & Neddermeyer and Street & Stevenson had identified in their cloud-chamber photographs a particle of mass intermediate between that of the electron and proton. Robert Serber and Robert Oppenheimer suggested that it was the theoretical particle predicted by Hideki Yukawa in 1935 as the carrier of the short-range strong nuclear force that binds the protons and neutrons in the nucleus. According to Yukawa's theory, his heavy quantum should have a mass of about 200 times the electron mass. For this reason such a particle was later called a “meson”. In the same paper, Yukawa suggested an alternative form of Enrico Fermi's beta-decay theory and assumed that the meson should be unstable and disintegrate spontaneously into an electron and an antineutrino, or positron and neutrino, providing a mechanism for nuclear beta decay. It was soon shown, by delayed coincidence experiments with counters, that the mean lifetime of such cosmic ray mesons - that Anderson and Neddermeyer had named “mesotrons” - was of the order of 10-6 s, a figure which was also roughly in accord with the expectations of Yukawa.
Physicists were now facing the new challenge of investigating the nature of the mesotron of cosmic rays and for the first time they were dealing with the phenomenon of spontaneous instability of a free elementary particle. Establishing the reality of such a process and the accurate determination of the mesotron’s mean life became one of the outstanding problems of cosmic-ray research, as particle physics was taking shape, emerging as a new field from the confluence of nuclear physics, cosmic-ray studies and quantum field theory. In a series of experiments performed with different collaborators and different techniques during the period 1939-1942, Bruno Rossi gave definitive proof of the mesotron decay, demonstrating the relativistic dilation of the lifetime of mesotrons in flight, and culminating in the first precise measurement of the mean life of mesotrons at rest.
A first hint on the real nature of the meson of cosmic rays was revealed during the war by a series of elegant experiments performed by the Italian team formed by Marcello Conversi, Ettore Pancini and Oreste Piccioni (CPP). The Yukawa quanta were expected to undergo strong interaction with atomic nuclei, and although numerous examples were known where mesotrons had traversed metal plates in cloud chambers, no example had ever been observed of their interaction “in flight”. Using a system of counters and magnetic lenses, devised by Rossi in 1930, for the separation of negative and positive mesotrons, the Italian team was able to observe the separate fates of negatively and positively charged mesons coming to rest in various materials. S. Tomonaga and G. Araki had calculated that negative mesons passing through matter would be more likely to be captured by nuclei than to disintegrate spontaneously, while for the positives the capture probability would be greatly reduced by the potential barrier.
The Italians had confirmed that the decay rate in iron for negative particles was actually compatible with the Tomonaga-Araki prediction. However, during their last experiment, they observed that negative mesons stopping in carbon - a lighter element - always, or nearly always, decayed spontaneously instead of being captured by the carbon nucleus, as would be expected. For the case of carbon, decay electrons were observed with a rate very similar to the rate measured with positive mesons, demonstrating that they could not be the nuclear quanta postulated by Yukawa, as Enrico Fermi, Edward Teller and Victor Weisskopf immediately clarified. There were about 12 orders of magnitude difference between the predicted capture time for a negative Yukawa particle and the results of the experiment for negative particles. They concluded that the CPP experiments implied that negative mesons are captured into states of low energy round the capturing nuclei, where they spend an appreciable fraction of their time in the nucleus itself. Such a behaviour is impossible for a strongly interacting particle which should interact in a time of the order of the characteristic nuclear time: viz: about 10-23 s. Such was the impact of the CPP experiment that much later Luis Alvarez remarked in his Nobel Lecture, that in his opinion this landmark experiment had inaugurated the modern era of particle physics.
The announcement of this unexpected behaviour immediately prompted Bruno Pontecorvo to put forward a bold hypothesis: “the probability (about 10-6 s) of capture of a bound negative meson is of the order of the probability of ordinary K-capture processes, when allowance is made for the difference in the disintegration energy and the difference in the volumes of the K-shell and of the meson orbit.” Pontecorvo's intuition that both the process of meson and of electron capture must be due to the same interaction, the weak interaction, was rather ignored at the moment. Soon after, many physicists provided a firm basis to the universal character of the weak interaction, capable of describing simultaneously nuclear beta-decay - as Fermi had done in 1934 - as well as the capture and decay of the “meson of cosmic rays”, whose real nature would soon be revealed by Powell's group’s experiments with improved nuclear emulsions.
The discovery of the pion
Throughout the war years, Powell continued the analysis of the emulsions from scattering experiments at the Liverpool cyclotron. These emulsions provided valuable data on, for example, the scattering of neutrons by uranium, and the importance of the emulsion technique - and of particle accelerators - soon became known to the UK government. Towards the end of 1945, the Ministry of Supply set up two panels: one, chaired by Blackett, to plan accelerator building and the other, led by Joseph Rotblat, to support and encourage industrial companies such as Ilford and Kodak to produce more sensitive nuclear emulsions. At the same time, the optical industry was encouraged to produce better precision microscopes to examine the developed photographs.
In 1945, Powell's group had been joined in Bristol by Giuseppe Occhialini who had returned to England from Brazil before the end of the war and was soon followed by his student Giulio Lattes. During the war years, Occhialini had devised a new kind of counter as well as new methods for recording the arrival of cosmic ray particles. At the same time, he had performed studies on the beta spectrum of rubidium in order to throw light on the different theories of beta decay. He thus had a great experience both in the field of nuclear physics and in cosmic ray studies.
Occhialini was enthusiastic about the potential of the photographic method and made approaches to Ilford Ltd with a view to improving the recording properties of emulsions. Although Powell's group had already shown that reliable range measurements could be secured, tracks were only distinguishable if the number of developed grains along the track was sufficiently numerous. This depended upon the number of ions knocked out of atoms by a particle per unit length of its path, a number which gets lower the higher the velocity of the particle. In effect, as matters then stood, they could detect particles only when they were of relatively low speed, such as they encountered in the experiments on the cyclotron, but most of the particles with speeds approaching that of light, which are the most significant in cosmic radiation, eluded them. From collaboration with Ilford, new kinds of plates were developed specifically dedicated to nuclear research. The new emulsions were prepared with a very substantial increase in the concentration of silver bromide, in order to have great number of grains per unit volume of emulsion. When the new emulsions were exposed and developed it was clear that a remarkable improvement in recording properties had been achieved.
Occhialini immediately took a few small plates coated with the new emulsions and exposed them at the French observatory in the Pyrenees at the Pic du Midi at an altitude of 3,000 m. When they were recovered and developed in Bristol it was immediately apparent that a whole new world had been revealed. The tracks were so packed with developed grains that it appeared almost like a solid rod of silver and the whole tiny volume of emulsion appeared under the microscope to be crowded with disintegrations produced by fast cosmic ray particles. The richness of the resulting tracks made Powell recall in his autobiography: “It was as if, suddenly, we had broken into a walled orchard, where protected trees had flourished and all kinds of exotic fruits had ripened in great profusion.”
Among the earliest disintegrations which attracted their attention was one from which a particle was ejected which, at the end of its short range, seemed to be crossed by a short straight track. This raised one of the technical difficulties inherent in the method. Whereas the tracks recorded in the expansion of a cloud chamber, or of a bubble chamber, are certainly contemporaneous within a period of the order of a few milliseconds at the instant of the expansion, all that one can assert of an emulsion is that the tracks were recorded at some time between manufacture and development. It is in fact a great advantage of the photographic method, and also one of its limitations, that it is continuously sensitive, from the time the emulsion is dried during manufacture, up to the moment that the plate is developed. The problem presented by the “hammer tracks” was therefore whether the “handle” and the “head” of the “hammer” were due to related particles, or whether they represented an accidental coincidence of events quite unconnected with one another. This problem repeatedly occurred in the subsequent development of the method and was of crucial importance in a situation where far-reaching conclusions might be drawn from a single, or a very few, isolated observations. At the same time it is easy to show that the probability of a chance, but precise, juxtaposition of tracks is extremely improbable with the density of tracks in emulsions that were commonly employed. Nevertheless before drawing important conclusions, it was essential to observe great caution in scrutinising the tracks. They were greatly puzzled by this hammer track until Powell recalled a decay process of Lithium-3 artificially created in nuclear disintegrations that would account precisely for the characteristics of their track. Subsequently many other events of a similar nature were observed which gave them confidence in the correctness of this interpretation, but during this early period they lacked the confirmatory evidence which the track of the decay electron would have provided, for the new emulsions were not sensitive enough to give recognisable electron tracks.
In the lively atmosphere of enthusiasm and anticipation produced by these new observations, an exciting event attracted their attention. Associated with a small disintegration, there appeared to be a particle, a meson of mass about 200 times that of the electron, which had reached the end of its range at the point where a disintegration occurred. There were only two possible explanations of the observed tracks. Either the particle had come to rest at a point that, by chance, coincided with that of a completely independent disintegration; or the tracks were related in which case the sequence of events was unambiguous. Just at this time D. Perkins, at the Imperial College in London, who had independently been making similar experiments with the new emulsions exposed in a Royal Air Force plane flying at high altitudes, found an “event” with closely similar characteristics. The observation of two “events” of a similar nature seemed to exclude completely the possibility of a chance juxtaposition of unrelated tracks and it appeared certain that they were observing the consequences of the capture of a negative meson by a nucleus of an atom in the emulsion, and its resulting disintegration. The observation of disintegrations produced by slow mesons gave a dramatic demonstration of the transformation of mass into energy since the whole mass of the meson disappears and no kinetic energy contributes to the disintegration.
Very soon, in spring of 1947, one of the scanners who carefully examined the developed plates, found something completely different, an “event” which puzzled her. It appeared that a slow-moving meson had come to rest, and from the point where it had stopped, a second meson had emerged. This second meson had passed out of the emulsion before stopping, but it was only moving slowly when it escaped from observation, and thus its true range can have been only a little greater than the observed length of track. Powell and a young Bristol graduate, Hugh Muirhead, immediately recognised that this event was due to two mesons, not just one. The observers were asked to keep a close look-out to similar events, and a day later a second example was found which seemed precisely similar in significance to the first, but with the advantage that the secondary meson, diverging from the end of the range of its parent, was this time arrested in the emulsion so that its precise range could be determined. They were immediately struck to find that the two secondary mesons in the different events appeared to be ejected with equal, or closely similar, velocities. The similarity in range of the two secondary particles indicated that they were witnessing two-body decays: in both events a heavier, primary meson had decayed into a secondary, lighter meson and a neutral particle. This second event gave them great confidence that they were dealing with an important phenomenon, not a chance juxtaposition of unrelated tracks. If the chance of one such association was small, the chance of finding two representing closely similar phenomena was entirely remote.
Lattes, Muirhead, Occhialini and Powell published their findings in Nature on 24 May 1947. They suggested that what they had found was a new mode of meson decay, but added that further evidence was needed to justify such a radical conclusion. But what kind of process was involved? Powell recalled that “It seemed certain that a meson had reached the end of its range and that a second meson had been ejected from the point of arrest. But how long the first meson rested at the end of its range before the secondary was ejected was entirely uncertain, and although there were indications that the secondary emerged with a definite energy such a view at this stage could not be asserted. The most obvious explanation of the process was that the primary meson was more massive than the secondary; that those we were observing were positively charged and that on reaching the end of their range they transformed spontaneously into secondaries of the same charge, the kinetic energy being provided by the equivalent mass which disappeared in the process. It then followed from the principles of conservation of momentum and energy, that if the secondary meson was indeed always being ejected with the same energy, only a single neutral particle was recoiling from it at the instant of decay of which the track would, of course, remain unobserved.” Under these assumptions, if the secondary were identified as the penetrating particles of the cosmic radiation, whose mass was known to be about 200 electron masses, and if the neutral particle was a neutrino or a gamma-ray of zero-rest mass, then it was easy to show from the conservation laws that the mass of the primary must be about 265 electron masses. These tentative conclusions were later substantiated and illustrate the power of the method to permit far-reaching conclusions on the basis of a single, or of a very few observations.
But it was clearly crucial to find more examples of the process, so that the constant velocity of ejection of the secondaries could be established and so that favourable examples of flat tracks could be found which would permit estimates of the masses of both the primary and the secondary mesons to be made by the rough methods which were then available. Therefore, armed with further emulsion plates, Lattes travelled to Mount Chacaltaya in the Bolivian Andes, which lies 5,600 m above sea level - much higher than the Pic-du-Midi. These plates yielded 10 more two-meson decays. In all cases, the secondary meson came to rest in the emulsion with a range of about 600 micrometres. The new events confirmed that the secondary mesons do indeed commonly appear with kinetic energies constant within a narrow range, and they were confident they were dealing with two different particles with masses of the order of 200 and 260 electron-masses, respectively.
There had been previous speculations by theoreticians that there were two kinds of mesons in nature, notably by Sakata in 1944 and later by Marshak in 1947, but the Bristol team had known nothing of them. These speculations were based upon what seemed an irresolvable contradiction in the behaviour of the cosmic ray mesons, which constitute the well-known penetrating component of the cosmic radiation. They were believed to be created in the high atmosphere when the incoming protons, which form the greater part of primary flux of the cosmic rays approaching the earth from outer space, collide with the nuclei of the atoms of the air. Such copious production in such interactions implied a very strong interaction with nuclei. On the other hand, the CPP experiment had clearly shown that the negatively charged mesons, when they are captured by light elements such as carbon, decay with mean lifetimes approximately equal to the value found when the particles are free. The puzzle of the copious production and weak nuclear interaction of cosmic-ray mesons could thus be resolved by the discovery of the two types of mesons revealing that there was a strongly interacting meson which transformed spontaneously into a weakly interacting and relatively long-lived meson.
On October 4, 1947, Lattes, Occhialini and Powell published in Nature the result of their observation of meson tracks found in plates exposed at the Pic du Midi and at Chacaltaya in the Bolivian Andes at 5,500 m. They concluded that they had seen a primary meson, which they named the “pion” decaying into a secondary meson, which they called the “muon”.
As it turned out later, the primary particle, designated by the Greek letter pi by Lattes, Occhialini and Powell, is just the lightest member of the family of strongly interacting mesons, which are composed of various combinations of quark-antiquark pairs. They are produced directly in nuclear reactions but they rapidly decay, when in flight in the atmosphere, to produce mu-mesons, as the mesons of cosmic rays were now renamed. They proceed nearly in the line of motion of the parent pions and form the penetrating component of the cosmic rays. They also found that there were events in which a single meson produced a nuclear disintegration. They therefore concluded that there were in fact two types of pions - positive and negative. In the two-meson events, a positive pion was repelled by the positively charged nucleus before decaying into a muon. In the single-meson events, a negative pion was captured by the nucleus, causing the nucleus to disintegrate.
Their early reports on these results were received with a certain very proper reserve by other physicists. There was hesitation in giving credence to such important conclusions on the basis of a method with which most physicists had had no experience. Further, their findings were supported by mosaics of micro-photographs, for as a track wanders at changing depths in the emulsion it passes out of focus; a succession of overlapping photographs must be taken with appropriate settings in order to obtain enough to construct a mosaic which gives a picture of the whole event at high resolution. However, with ten or twelve secondary meson tracks measured, it was possible to begin to plot the distribution in the values of their range and obtain the evidence that they represent a group homogeneous in velocity.
By 1948-1949, Occhialini and his wife Constance Dilworth had perfected a method for processing emulsions as thick as 600 micrometres, which meant that much longer particle tracks could be recorded. Accordingly, Ilford and Kodak succeeded in starting commercial production of a new kind of emulsion. They revealed the complete decay sequence of a positive pion into a muon into a positron, as well as high-energy nuclear disintegrations that produced showers of relativistic particles - mostly positive and negative pions. Powell also obtained clear evidence for neutral pions, which decay into two gamma rays that produce electron-positron pars.
However, they knew that fast electrons (and positrons) moving at velocities near that of light failed to give recognisable tracks, so they continued to urge the photographic industry to increase the sensitivity of the emulsions in order to record the wealth of phenomena that they suspected must be occurring in their emulsions. In the course of about 18 months, Kodak produced the first electron-sensitive emulsion that allowed them to find an example of the track of a positive pion that had first decayed with the emission of a muon, the muon subsequently transforming into an electron of which the track was clearly distinguished; other examples quickly followed. The electron-sensitive emulsions also allowed them to decide whether the neutral particle that recoils from the muon when the pion decays is a gamma ray or some form of neutrino. The study of a large number of positive pion decays led to the conclusion that the neutral recoiling particle was rarely, if ever, a gamma ray. It was therefore reasonable to attribute it to some form of neutrino, possibly, but not certainly, identical with the neutrinos of beta-decay. In 1959, Bruno Pontecorvo proposed to perform an experiment using a high-energy accelerator in order to establish whether the muon neutrino is indeed different from the electron-neutrino. Melvin Schwartz had also independently envisaged the possibility of producing an intense neutrino beam from the decay of pions, particles copiously produced when a proton beam of GeV energy hits matter. The high-energy neutrinos could then be used as a probe to investigate the weak interactions. A decisive experiment was realised in 1961 by Leon Lederman, Schwartz, and Jack Steinberger at the Brookhaven Alternating Gradient Synchrotron with beams of neutrinos produced in association with muons in the decays of charged pions and kaons. They obtained a number of convincing events in which the neutrino beam induced the production of a muon, but never an electron. For having proved the existence of a type of neutrino associated with the muon, distinct from the neutrino produced in beta decay, they were jointly awarded the Nobel Prize for Physics 1988 “for the neutrino beam method and the demonstration of the doublet structure of the leptons through the discovery of the muon neutrino.” In confirming the reality of this difference, the experiment shed a deep insight on the fundamental features of the weak interactions and the riddle that the existence of the muons and different neutrinos poses. We now know that the muon is just one of six weakly interacting members of the lepton family.
Such was the impact of the pion discovery, the first major discovery of the post-war era, that Powell was awarded the Nobel Prize in Physics 1950 “for his development of the photographic method of studying nuclear processes and his discoveries regarding mesons made with this method”.
In showing the potential of cosmic-ray studies using the emulsion technique, the solution to the mesotron riddle opened the door to the discovery of many other fundamental particles. It was a period rich with discoveries for cosmic-ray physicists. Mesons heavier than the pion had already been seen at Manchester by George Rochester and Clifford Butler, two young physicists of Blackett's team. At the end of 1946, they discovered two peculiar forked traces in cloud chamber photographs. Blackett was very excited, and immediately recognised it as a new phenomenon. Measurements showed that the arms of the V were the tracks of positive and negative particles of large mass, excluding an electron-positron pair. Blackett provided some of the key arguments that convinced Rochester and Butler that these V-particles - having a mass of about 1000 times the mass of an electron - were in fact new neutral particles decaying to two charged particles. They were the first example of what would be known as “strange particles”. A second even more convincing event was found. This time it was a positive unstable particle decaying to one charged particle and one or more neutral particles. Using the Kodak emulsions, Powell's team found an event that showed a meson with a mass of about 985 electron masses decaying into three charged pions, as well as two clear examples of mesons whose mass was estimated to be about one thousand electron masses decaying into single charged particles. These examples opened up the enormous field of “strange particle physics” at a time when cosmic rays were still the main source of high energy particles.
The proliferation of these many different decays caused a great confusion that was only resolved with the advent of the new generation of accelerators. Later it became clear that these events - and those of Rochester and Butler - represent different decay modes of what are now called K-mesons. The identification of the decay modes of the K-mesons and hyperons, the precise measurement of their mass, and the study of their interactions had an important bearing on the formulation of the hypothesis of associated production of kaons and hyperons, and the introduction of the strangeness quantum number, by Pais, Nishijima and Gell-mann.
In the meantime, to obtain events at even higher energies, Powell and his Bristol group embarked in 1949 on a programme of balloon flights to expose emulsions high in the atmosphere to study the particles produced by collisions of the primary particles of cosmic rays. Early flights were carried out from the university sports ground, but at the end of 1951 Powell suggested collaboration with a large number of European laboratories in order to increase the statistics of the rather rare events under study as much as possible. To organise distant expeditions on a useful scale, international cooperation of many institutions was in fact necessary. The plates obtained would then be split among the various laboratories for microscope scanning. A decision was also taken to remove the site of balloon-flying operations to the Mediterranean in order to obtain better and more stable weather conditions allowing longer flights and the recovery at sea of the emulsion stacks and other equipment when they came down by parachute. Naples and Cagliari were chosen as the most suitable bases. The very large stacks of emulsion carried in these Italian flights were divided between the many participating universities. One of the most important of these stacks - the so-called G-stack (G = gigantic) - allowed a systematic analysis of the various decay modes of charged K-mesons.
The race to achieve new result was a race against time because large accelerators, like the Brookhaven Cosmotron, were starting to be used in the United States. The study of K-meson decays were the last important contributions of cosmic ray experiments to particle physics, marking the end of a period during which discoveries of fundamental importance were still made with the simplest apparatus. The British proposal took shape within the context of the setting up of CERN, which supported three international expeditions with the goal to create a spirit of cooperation. The international collaborations that grew up between the various emulsion groups were the forerunner of what was to happen later around the big high-energy particle accelerators, which gradually replaced cosmic-ray experiments in the study of elementary particles. Such cooperation on an international scale prepared in fact the setting-up and running of the CERN organisation, in which Powell played a large part.
Evidence for the pion decay and the study of K mesons were definitely opening the gates to the era of modern particle physics. The impact of the discovery of new fundamental particles was profound. It stimulated the building of a new generation of accelerators with which to probe the newly discovered domain of sub-nuclear physics. They went into operation during the early 1950s and soon took over from cosmic rays as a source of mesons. In the mid-1950s, the emergence of GeV accelerators was thus splitting the cosmic-ray community into astrophysicists and particle hunters. Some of them turned to work with accelerators, others began to apply themselves to cosmic ray problems different from those that had been in the foreground in earlier years. Attention was attracted to the new possibilities offered by the beginning of the space era at the end of the 1950s. Cosmic-ray physics evolved into two new disciplines, high-energy elementary particle physics and cosmic-ray astrophysics.
As accelerators and bubble chambers took the place of cosmic rays and nuclear emulsions, Powell's activities turned more towards formulation of scientific policies and the social responsibilities of scientists in which he strongly believed. At that time fundamental particle research entered the era of giant accelerator machines too expensive for any one nation in Europe to develop. From his position as a leader in European collaborative research, Powell then played a major role in the establishment of CERN, the European laboratory for particle physics in Geneva.
The award of the Nobel Prize allowed him a wider audience for his ideas on the need for international collaboration in science and the abolition of nuclear weapons. He was importantly involved in the discussions leading up to the Russell-Einstein declaration of 9 July 1955 and was one of the eleven original signatories. The manifesto, which called upon scientists to “assemble in conference to appraise the perils that have arisen as a result of the development of weapons of mass destruction,” led in 1957 to the foundation of the Pugwash Movement, with Powell among the founding members. He presided at the plenary meetings of the First Pugwash Conference and became Chairman of its executive, the Pugwash Continuing Committee, in 1967. Through meetings and projects that bring together scientists, experts, and policy makers, Pugwash focuses on those problems that lie at the intersection of science and world affairs. The periodic general Pugwash Conferences on Science and World Affairs have since then pursued the goal to seek the elimination of all weapons of mass destruction, to reduce the risk of war especially in areas where such weapons are present and may be used, and to discuss new scientific and technological developments that may bring more instability and heighten the risk of conflicts. It was in recognition of its mission to “diminish the part played by nuclear arms in international politics and, in the longer run, to eliminate such arms” that the 1995 Nobel Peace Prize was jointly awarded to Pugwash and its co-founder, Joseph Rotblat.
Bibliography
Frank F.C. and Perkins D.H. (1971) Cecil Frank Powell. 1903-1969. Biographical Memoirs of Fellows of the Royal Society 17: 541-563
Lock O. (November 1997) Cecil Powell: pions, peace and politics. Physics World: 35-40
Powell C.F. (without date) Fragments of Autobiography. Autobiographical fragment covering the early period of Powell's career available at http://www.bristol.ac.uk/physics/media/histories/12-powell.pdf