Laser
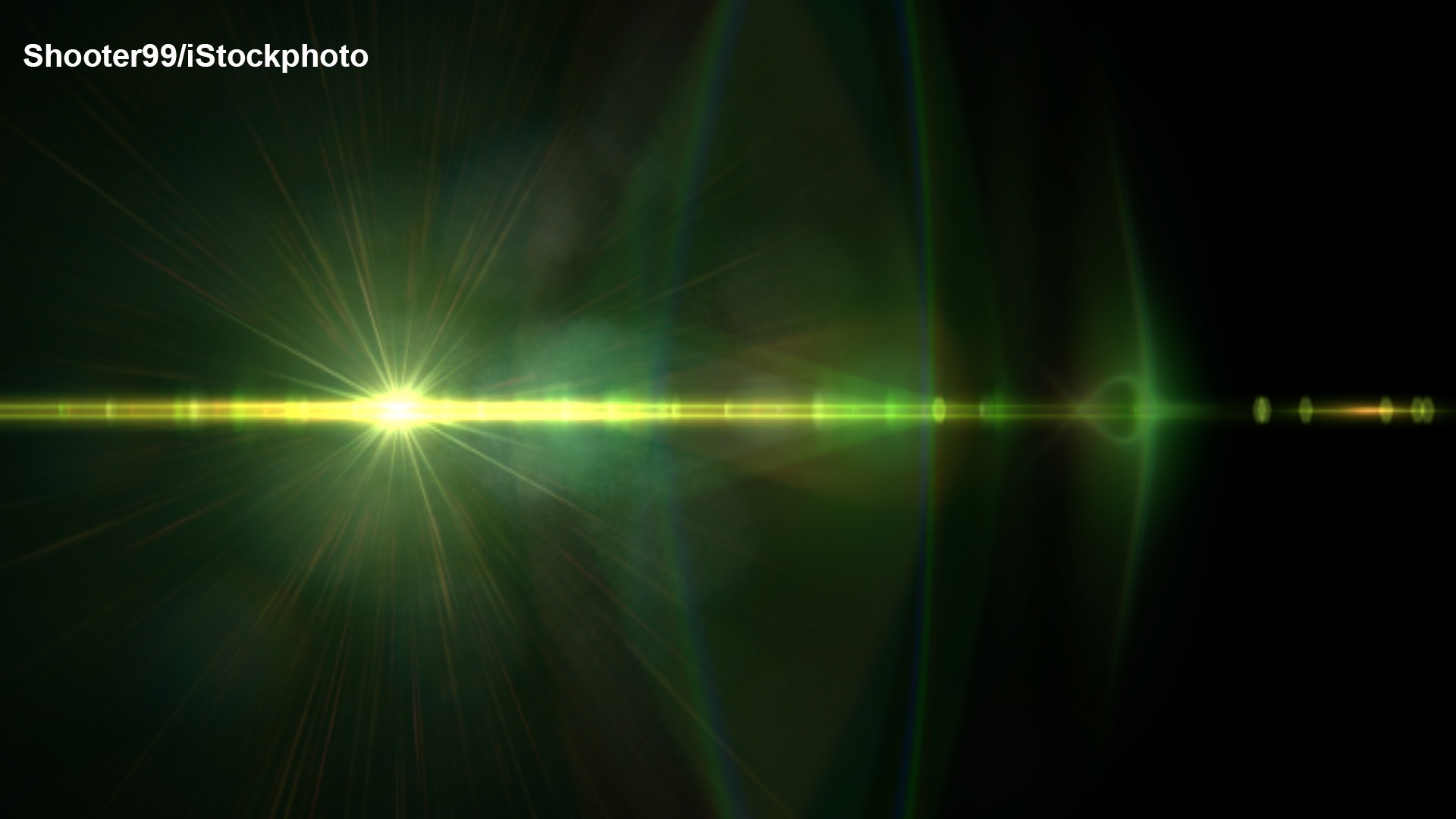
by Joachim Pietzsch
Laser light is made up of photons like sunrays or the radiancy of a torch. And yet it is very different from natural or electric light. What distinguishes it and makes it one of the most impactful and versatile inventions of the 20th century? First of all, its coherence, as William Phillips, Nobel Physics Laureate 1997, explained when he first came to Lindau in 2001:
Coherence means that all light waves of a laser beam are moving precisely in step with one another. Their peaks and troughs do not cancel each other out. In contrast to the light emitted by an electric bulb, for example, all of the energy originally released by a laser source remains present in the beam and can exert an enormous power when being focused in space and time, as Nicolaas Bloembergen, Nobel Physics Laureate 1981, illustratively described:
Bloembergen and Phillips are two of 20 scientists who have received a Nobel Prize for their achievements in laser related research and development since 1964. 14 of them have attended the Lindau Nobel Laureate Meetings at least once, although not all them have lectured on a laser related topic. The 20th of these laureates is Stefan Hell, who was awarded with a Nobel Prize in Chemistry 2014 and will come to Lindau in 2015.
Einstein’s third alternative for radiation by matter
Even if the time of laser related Nobel Prizes spans only half a century as of today, the centenary of the theoretical foundation of the laser is imminent. Albert Einstein laid this foundation in two papers in late 1916 and early 1917[1], in which he tried to “clarify somewhat the still unclear processes of emission and absorption of radiation by matter” and “made a few hypotheses about the emission and absorption of radiation of molecules, which suggested themselves from a quantum-theoretic point of view”.[2] According to Bohr’s atom model, in which electrons are orbiting the nucleus on distinct energy levels, two radiation processes were well understood at this time: absorption, when an electron takes up energy to jump on an upper level, and spontaneous emission, in which an excited electron falls back to its ground state and radiates an energy quantum precisely corresponding to the difference between the two levels. By analyzing Planck’s radiation formula, Einstein showed that there must be a third possibility: “An excited atom can be triggered into releasing its extra energy and going back into the ground state, if it is nudged, as it were, by a passing photon. This process is called stimulated emission, and it only happens if the passing photon has exactly the same wavelength as the one that the atom is primed to radiate.”[3]
If photons stimulate atoms to emit more photons of the same wavelength by hitting excited electrons, a chain reaction starts, in which light is amplified. Such a chain reaction is the typical feature of a LASER, which is an acronym for Light Amplification by Stimulated Emission of Radiation. But why did more than four decades pass by between Einstein’s insight and the invention of the laser? Because physicists long regarded stimulated emission as a purely theoretical concept, unfit for practical application. They knew that under normal conditions, in which ground energy levels prevail, the energy of incoming photons is digested in absorption processes rather than harnessed for stimulated emission.
From theory to practice: “Twisting nature a bit”
“For every atomic or molecular downward transition that contributes a photon to a passing wave, there are even more at lower energy levels to absorb the same energy photons in upward transitions. So, if a substance is in thermal equilibrium, this process is a net looser. That is what the second law of thermodynamics directly implies. The material soaks up more photons than it surrenders”, as Charles Townes put it, the man who found a way to “twist nature a bit” while investigating the generation of millimeter microwaves for the purpose of improving atomic spectroscopy at Columbia University in New York in the early 1950s. “There is no inviolable requirement that all systems be in thermal equilibrium. If one were somehow to have a collection entirely of excited molecules, then, in principle, there would be no limit to the amount of energy obtainable.”[4] Based on this thought, he devised the sketch of an “apparatus for obtaining short microwaves from excited atomic or molecular systems”[5] in his notebook on May 11, 1951. Townes must have felt the importance of this entry, because he let it be witnessed by a statement of his post-doc Arthur Schawlow. Independently from Townes and his group, Nikolay Basov and Aleksandr Prokhorov at the Lebedev Institute in Moscow solved the problem of creating a “collection entirely of excited molecules”, or inverted population, in order to amplify microwaves. All three scientists shared the Nobel Prize in Physics 1964 “for fundamental work in the field of quantum electronics, which has led to the construction of oscillators and amplifiers based on the maser-laser principle.” In his first lecture in Lindau in 1979, Basov described the essential clue for the creation of lasers:
Five orders of magnitude away: From maser to laser
It is noteworthy that Basov, Prokhorov and Townes did not construct the first laser, but rather invented the maser, i.e. microwave amplification by stimulated emission of radiation. Light has a 100.000-fold higher frequency than microwaves, so that it was quite a huge step from the maser to the laser in terms of technical prerequisites and operation conditions. The method of optical pumping for obtaining inverse populations decisively supported this step. It had been proposed by Alfred Kastler and “played an important part in the development of the laser”[6], as the Royal Swedish Academy of Sciences acknowledged when Kastler was awarded with the Nobel Prize in Physics 1966 “for the discovery and development of optical methods for studying Hertzian resonances in atoms". Kastler was a frequent guest in Lindau and attended the Nobel Laureate Meeting six times between 1968 and 1982. While the three 1964 laureates and Arthur Schawlow all contributed to the development of the laser, the acronym „Laser“ was coined by graduate student Gordon Gould in November 1957. In fact, Gould made such important inventions in the field that he was issued forty-eight laser-related patents after a legal patent battle that did not end before 1987, and subsequently was elected to the National Inventors Hall of Fame. The first working laser device, however, was constructed by Theodore Maiman[7]. Using the chromium atoms of a ruby crystal as the medium, he made it operate on May 16th, 1960, at the Hughes Research Laboratory in California, based on the technique proposed by Schawlow and Townes[8]: The medium is contained within an optical cavity with a pair of mirrors at either end. Its atoms are excited by optical pumping and exposed to light radiation. The photon cascade, which results from stimulated emission, bounces back and forth between the mirrors, so that it is more and more amplified. One of the two mirrors is partially reflective and partially transparent. This is the so-called output coupler through which the coherent light that has been produced within the cavity (or resonator) is sent out as a laser beam.
A solution sets out to find its problems
Neither scientists nor engineers were really prepared for the first laser, as Charles Townes remembered more than four decades later. “Many people said to me—partly as a joke but also as a challenge—that the laser was ‘a solution looking for a problem.’ But by bringing together optics and electronics, lasers opened up vast new fields of science and technology.”[9] Soon, lasers found their first very successful applications for calibrating purposes in the construction trade. Not much later they began to be used, inter alia, for drilling holes (not least in the diamond industry), for hardening surfaces (in the body construction of cars for example), for treating refractive errors and repairing the retina in eye surgery and as means to both cut and weld living tissue in general surgery with a laser scalpel.
One also learned to use them for improving the precision of infrared astronomy. This explains why Charles Townes became a pioneer in this field. When he gave his first lecture in Lindau in 1971, he consequently spoke about his current research activities in astrophysics, namely about his discovery that outer space amazingly contains complex molecules. In this context, he also introduced a naturally occurring maser:
Alexandr Prokhorov, on the other hand, extensively discussed a special feature of strong laser beams when he talked about “some aspects of quantum electronics” in 1968, namely the phenomenon of self-focusing:
The magic of non-linearity
The fact that a powerful beam of light increases the refractive index of the medium, which it crosses and therefore generates its own collective lens while propagating is a special case of non-linearity. In a nonlinear medium, the usual relationships of optics do not apply. The polarization of light is not proportional to the amplitude of its electric field, for example. This effect only becomes observable at very high intensities of light, however. The invention of the laser therefore was a prerequisite to establish the field of nonlinear optics.
The result of Peter Franken’s pioneering experiment – the (partial) transformation of red into ultraviolet laser light – was due to a second-harmonic generation or frequency doubling. Nicolaas Bloembergen and Peter Pershan first formulated this insight mathematically in 1962. Revisiting Maxwell’s equations, they described the behavior of light waves at the interface between a linear and a nonlinear medium, generalized the classical laws of optics, namely the laws of reflection and refraction, and thus laid a theoretical foundation for non-linear optics.[10] Bloembergen made many more contributions to non-linear optics, and so did Arthur Schawlow with whom he shared a Nobel Prize in Physics 1981 “for their contribution to the development of laser spectroscopy”. The latter succeeded in eliminating the broadening of spectral lines that were caused by the Doppler effect due to the motion of atoms and thereby brought atomic spectroscopy to a hitherto unknown level of precision, the first succeeded in mixing three laser beams so that a fourth one is generated. This made it possible to generate laser light far outside the visible spectrum, both in the infrared and the ultraviolet range. A special form of this four-wave mixing method is “Coherent Anti-Stokes Raman Scattering” – a non-linear technique that is sensitive to the nuclear vibrations in chemical bonds and “has been applied in studies of widely differing kinds - all the way from optimization of combustion processes in motorcar engines to the study of element transport in biological tissues“.[11] The laser and its associated non-linear techniques also turned out to be the tools that were necessary to realize holography, which had been conceived by Dennis Gabor already between 1948 and 1951. „For his invention and development of the holographic method“, Gabor consequently was awarded with the Nobel Prize in Physics 1971. As a member of the „Club of Rome“ he came to Lindau in the following year to talk about the „predicament of mankind“.
The triumphal procession of semiconductor lasers
Many materials can act as laser media and several types of lasers are known. The most impactful of these types is the semiconductor laser whose principle Zhores I. Alferov and Herbert Kroemer proposed independently of each other in 1963: An electric current induces an inverse population state within a thin semiconductor layer that is sandwiched between two other heterostructures and triggers the upright emission of laser light from that layer. When such laser diodes became able to work continuously at room temperature around 1970, the laser’s triumphal procession in daily life applications, consumer electronics and information technology began: The first bar code scanners were installed at supermarket checkouts in 1974, the laser disc player was introduced in 1978, laser pointers and laser printers became popular and, of course, the read-out of densely stored digital information and the very fast transmission of signals via fiber-optic cables became possible and with it the broadband communication facilities that we are taking for granted in the era of the world wide web. Alferov and Kroemer shared one of the Nobel Prizes in Physics 2000, which was awarded to them for “basic work on information and communication technology“. The other prize was awarded to Jack Kilby “for developing semiconductor heterostructures used in high-speed- and optoelectronics”. Both Alferov and Kroemer lectured in Lindau for the first time already in the following year. Charles Kao, however, who received a Nobel Prize in Physics 2009 “for groundbreaking achievements concerning the transmission of light in fibers for optical communication” did not come to Lindau so far.
In his first Lindau lecture, Herbert Kroemer vividly recalled his eureka moment:
Zhores Alferov spoke about the state of the art and future trends in heterostructure research:
The foundations for most of the applications that have made the laser popular and become indispensable for our present age were laid already during the 1960s. The research results that were achieved during the following decades and have been acknowledged by Nobel Prizes in 1997, 1999, 2001, 2005 and 2014 did not have such immediate consequences for our life style, but rather propelled basic science into new dimensions, namely in the fields of precision measurement, matter transformation and both temporal and spatial resolution.
A stick to measure a millionth billionth of a second pulse
John Hall and Theodor Hänsch shared one of the Nobel Prizes in Physics 2005 “for their contributions to the development of laser-based precision spectroscopy, including the optical frequency comb technique”. The necessity to develop such a high precision spectroscopy arose during the process of redefining the length of a meter. At an international conference in Paris in October 1983 the meter was redefined by coupling it to the second and determining it as the reciprocal value of the velocity of light, respectively: One meter is the distance light travels in 1/299,792,458 second. John Hall was greatly involved in this redefinition process and shared the excitement about it in his lecture in Lindau in 2012:
(00:08:59 - 00:14:19)
The new definition of the meter, however, soon turned out to be unusable for most practical purposes. The frequency of light is close to 1015 Hertz. The frequency of the cesium clock on which the definition of a second is based (9,192,631,770 oscillations of the radiation inducing the hyperfine transition in 133Cs ) is approximately 105 times slower. Determining the wavelength of a frequency-stabilized laser expressed in the new meter unit therefore was so complicated that only a few highly specialized laboratories in the world could do it. A new method for measuring optical frequencies had to be found. In two publications in the late 1970s[12], Theodor Hänsch and coworkers first described the principle of this method, which he and John Hall subsequently developed to perfection, namely the optical frequency comb technique that made it possible to measure frequencies with an accuracy of fifteen digits. In his lecture in Lindau in 2013 Theodor Hänsch illustratively explained this technique:
(00:02:09 - 00:07:22)
Observing chemical reactions in real time
With the help of extremely short femtosecond laser pulses it also became possible to observe how atoms in a molecule behave during a chemical reaction. A femtosecond relates to a second as a second relates to 32 million years! Ahmed Zewail initiated this new research field of femtochemistry in the late 1980s. The results of his work were so important that Arthur Schawlow introduced them to his Lindau audience already in 1991:
„This type of investigation allows us to understand and predict important reactions“, the Royal Swedish Academy of Sciences stated, when it awarded Ahmed Zewail with the Nobel Prize in Chemistry 1999 „for his studies of the transition states of chemical reactions using femtosecond spectroscopy“, and compared his technique to using the „world’s fastest camera“ in order to obtain „slow motion“ pictures of molecular interactions.[13] In 2002, Zewail attended the Lindau Nobel Laureate Meeting to speak about „Chemistry and biology in new light“.
Creating the fifth aggregate state of matter
Slowing down the fast moving atoms in gases without letting them condense into liquids and then freeze into solids, is of great importance for basic research into the nature of matter. At their room temperature speed of about 4000 kilometers per hour the atoms and molecules of gases are hard to study. The application of laser light can slow their motion to a speed of less than one kilometer per hour. From 1985 on, Steven Chu, Claude Cohen-Tannoudji and William Phillips successfully developed techniques for such applications, an achievement, which jointly earned them the Nobel Prize in Physics 1997 “for development of methods to cool and trap atoms with laser light”. Their technique in turn formed the basis “for the achievement of Bose-Einstein condensation in dilute gases of alkali atoms, and for early fundamental studies of the properties of the condensates”, for which Eric Cornell, Wolfgang Ketterle and Carl Wieman shared the Nobel Prize in Physics 2001. Theoretically, the existence of such condensates, which in addition to plasma, gas, liquids and solids are regarded as the fifth aggregate state of matter, had been postulated by the Indian physicist Satyendra Nath Bose and Albert Einstein seven decades before their first realization in 1995. In Bose-Einstein-Condensates a large fraction of specific particles (bosons) convert to the lowest energy state and the atoms become coherent, which makes macroscopic quantum phenomena apparent. The coherence of the bosons in Bose-Einstein-Condensates can be compared to the coherence of photons in laser light, as William Phillips explained in Lindau in 2001, several months before the Nobel Prize concerning this matter was announced.
Transcending an asserted limit of spatial resolution
Life scientists generally prefer light microscopy to electron microscopy because it is the only way to non-invasively look into a living cell or tissue in three dimensions. Additionally, it offers the possibility to attach fluorescent markers to the molecules of interest. For a long time, however, it was taken for granted that light microscopy never could reach the resolution of electron microscopy. According to the “law” (as every textbook cited it) of the German physicist Ernst Abbe, the resolution limit of the light microscope was confined to half the wavelength of light, which amounts to approximately 200 nanometers. How does it come that nobody questions this law, Stefan Hell asked himself in the mid 1990s. It had been postulated 120 years ago, and in the meantime so much progress had been made in physics. “There should be one phenomenon”, Hell thought, “at least one that could be used to overcome this diffraction barrier.”[14] In quest of this phenomenon, he thoroughly revisited his textbooks – and found it in “The Quantum Theory of Light”: Laser light. It could be used, Hell reasoned, to separate molecules under the light microscope. Based on this idea, Hell invented stimulated emission depletion (STED) microscopy, in which he used two laser beams, one to stimulate fluorescent molecules to glow, the other to keep adjacent molecules dark. “You can see a lot by observing: Optical microscopy 2.0” , Steven Chu titled his lecture in Lindau in 2014, in which he explained the principle of STED microscopy:
(00:25:23 - 00:28:19)
A few months later, Stefan Hell received a Nobel Prize in Chemistry for his invention of STED microscopy. “Is there a limit?” he asked in his Nobel lecture on “nanoscopy with focused light”. “The name of the game is that we turn molecules off”, he replied and concluded that therefore “the resolution limit is the size of the molecules because this is the smallest entity you can separate”.[15]
As of today, Hell’s invention of STED microscopy is the latest Nobel Prize winning achievement related to the LASER. Most probably it will not be the last one. Not only because laser beams promise to help surpass even more supposed limits, but also because “there is still much to learn about the nature of light” – as one of the inventors of the LASER once formulated it in Lindau as a timeless challenge.
Footnotes:
[1] Einstein, Albert. Strahlungs-Emission und -Absorption nach der Quantentheorie [Emission and Absorption of Radiation in Quantum Theory]. Verhandl. D. Deutsch. Phys. Ges., 1916, Vol 18, pp. 318-323.
Einstein, Albert. Zur Quantentheorie der Strahlung [On the Quantum Theory of Radiation]. Phys. ZS., 1917, Vol. 18, pp. 121-128
[2] Einstein 1917, p. 122
[3] John Gribbin. In search of Schrödinger’s cat. Quantum physics and reality. London 1984, p. 134
[4] Charles H. Townes. How the laser happened. Adventures of a scientist. New York 1999, p. 56
[5] Ibd., p. 59
[6] http://www.nobelprize.org/nobel_prizes/physics/laureates/1966/press.html
[7] Cf. Maiman TH. Stimulated optical radiation in ruby. Nature 187, 493-494 (6 August 1990)
[8] Schawlow AL and Townes CH. Infrared and optical masers. Physical review 112, 1940-1949 (15 December 1958)
[9] Charles Townes. The first laser. In: Laura Garwin and Tim Lincoln (ed.). A century of Nature: Twenty-one discoveries that changed science and the world. Chicago University Press 2003, p. 107-112
[10] N. Bloembergen and P.S. Pershan. Light waves at the boundary of nonlinear media. Phys. Rev. 128, 606 - 622. 15 October 1962
[11] http://www.nobelprize.org/nobel_prizes/physics/laureates/1981/press.html
[12] Teets R, Eckstein J, and Hänsch TW. Coherent two-photon excitation by multiple light pulses. Phys. Rev. Lett. 38, 760 (1977)
Eckstein JN, Ferguson AI, and Hänsch TW. High-resolution two-photon spectroscopy with picosecond light pulses. Phys. Rev. Lett. 40, 847 (1978)
[13] http://www.nobelprize.org/nobel_prizes/chemistry/laureates/1999/press.html
[14] Cf. http://www.nobelprize.org/nobel_prizes/chemistry/laureates/2014/hell-lecture.html
[15] Ibd.
Additional Lindau Lectures on Laser
2004 Nicolaas Bloembergen: "Lasers in Peace and War".