Light & Optics
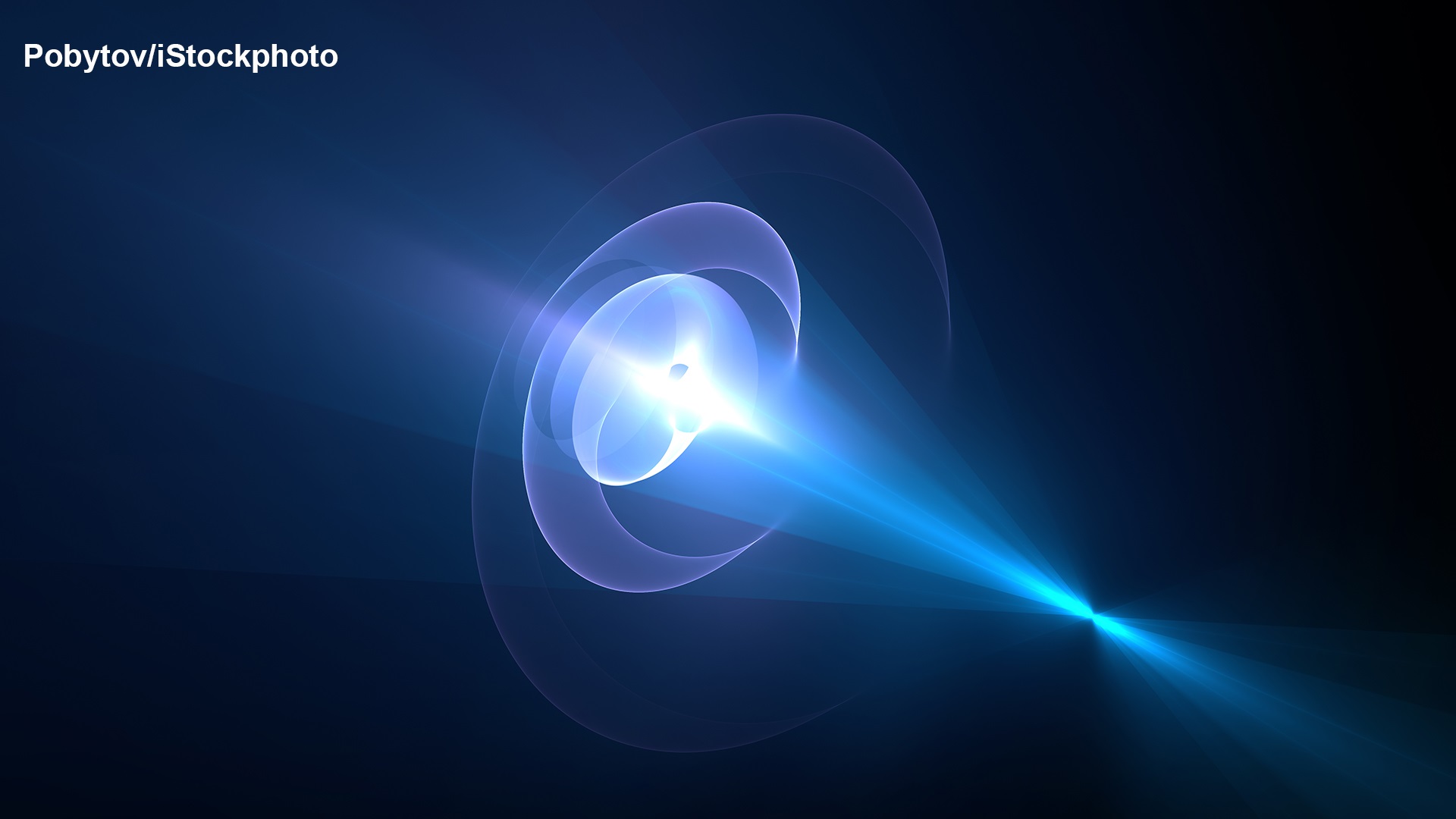
by Meeri Kim
A brief history of optics
While the earliest beliefs about light revolved around religion, ancient Greek philosophers were the first who sought to explain the true scientific nature of this phenomenon. Pythagoras thought that rays emanated from the eye and struck objects to create vision, whereas Epicurus proposed that light comes from sources other than the eye, reflects off objects, and enters the eye [1]. Later work by Euclid and Ptolemy revealed the basis for the field now referred to as geometrical optics, which describes light propagation in terms of rays [2].
The further understanding of light's properties such as straight-line propagation, reflection, and refraction led to new applications of lenses for things other than burning or magnifying glasses. In the centuries that followed, inventors produced the first vision-correcting glasses, compound microscopes, and telescopes [1].
In the 17th century, two competing models of light emerged. On one side, René Descartes and Christiaan Huygens described light as a propagating wave, while on the other side, Isaac Newton argued for a particle theory of light. In his seminal 1704 work Opticks, Newton postulated that light consists of a stream of corpuscles or particles, with particles from different colors of light having different masses. His model remained dominant until the 19th century, when studies confirmed that light is actually an electromagnetic wave.
But of course, the story doesn't end there. The dawn of quantum mechanics in the beginning of the 20th century overturned well-established notions about the nature of light once again. Quantum mechanics, a theory on the behavior of matter and light on the atomic and subatomic scale, was developed independently by Werner Heisenberg and Erwin Schrödinger. Paul Dirac, who was a regular guest at the Lindau Physics meetings, spoke about the early foundations of quantum mechanics in his 1965 lecture.
(00:00:20 - 00:01:20)
During his lecture at the Lindau Nobel Laureate Meeting in 2016, Theodor W. Hänsch spoke about how the work of Max Planck and Albert Einstein contributed to the discovery of a paradoxical wave-particle duality.
(00:04:22 - 00:05:59)
In particular, the photoelectric effect was a phenomenon discovered in 1887 that represented an interaction between light and matter that could not be explained by classical physics. The effect occurs when electrically charged particles are ejected from a material’s surface when it is illuminated with electromagnetic radiation. The maximum kinetic energy of the released particles was proportional to the frequency, not the intensity, of the light. Eventually, Einstein formulated a new corpuscular theory of light in 1905 that defined each particle of light, or photon, as containing a fixed amount of energy that depends on the light’s frequency. During his first Lindau meeting in 1962 – and his very last public lecture before passing away the same year – Niels Bohr discusses Einstein’s explanation of the photoelectric effect.
(00:18:43 - 00:20:15)
Is light a particle or a wave?
Ultimately, neither a classical wave model nor a classical particle model could hold up to experimental findings in the end, and both were found to be incorrect. For instance, take the case of Thomas Young's double-slit experiment in 1801, which initially served as evidence of light as a wave [3]. When monochromatic light passing through two narrow slits illuminates a dark screen, an interference pattern is observed due to the two overlapping light waves. But when the screen is replaced by a single-photon detector, each photon arrives whole and intact at one point — but over time, many photons create the interference pattern seen in Young's original experiment.
Quantum mechanics solved this paradox by interpreting the magnitude of the interference pattern at any one point as the probability of a photon arriving at that point. From there, the field of quantum optics was born, which studies the interactions between light and matter that depend on the superposition of quantum states according to the general rules of quantum mechanics. Roy Glauber, in a lecture and discussion at the Lindau Nobel Laureate Meeting in 2010, describes how quantum optics came about from Paul Dirac's quantum electrodynamics, the relativistic quantum field theory of electrodynamics.
(00:52:45 - 00:56:35)
Applications of light: Invention of the blue LED
These fundamental discoveries about light have enabled the creation of countless new technologies. Photonic applications impact just about every industry imaginable: consumer equipment, medical, military, industrial manufacturing, telecommunications, computing, and so on. Most of these devices use semiconductor light sources like light-emitting diodes (LEDs) and lasers. For instance, LEDs serve as indicator lights for consumer electronics, transmit signals from remote controls, light up traffic signals, and send data through telecom fibers [4].
Leo Esaki, who won the 1973 Nobel Prize in Physics for experimental discoveries regarding tunneling phenomena in semiconductors, spoke during his 1997 lecture about the importance of fundamental discoveries by scientists that contributed to the information technology revolution.
(00:07:07 - 00:08:35)
Twenty-four scientists have received a Nobel Prize for their achievements in laser- or LED-related research and development since 1964. One of the more recent Nobel-worthy achievements was made by Isamu Akasaki, Hiroshi Amano, and Shuji Nakamura for their invention of energy-efficient and environmentally-friendly blue LEDs [5]. Because an LED lamp requires less power to emit light as compared to incandescent bulbs, it has the potential to improve the quality of life for over 1.5 billion people around the world who lack access to electricity grids.
In his 2016 talk at the Lindau Nobel Laureate Meeting, Amano tells the audience how he initially thought blue LEDs would only be useful for electronics displays, and how new technology can lead to new applications.
(00:20:49 - 00:22:44)
Previous to their work, only red and green diodes existed — and without the addition of blue diodes, white light could not be created. In 1907, the first report of light being emitted from a semiconductor came a publication by Henry J. Round, an assistant of Nobel Laureate Guglielmo Marconi [4]. The significance of this finding wasn't realized until decades later, and in 1962, the first red LED was developed by Nick Holonyak, Jr. while working at General Electric. The first green LED followed several years later in 1974.
Naturally, several groups in the 1970s embarked on a quest to create a blue LED next. All their efforts failed, and the projects were subsequently abandoned. But Akasaki, Amano, and Nakamura kept working on the problem, and after many years of research, they created a high-quality gallium nitride crystal that finally enabled the invention of the coveted blue LED [5]. Akasaki and Amano showed off their bright blue diode in 1992, while Nakamura found success a year later in 1993.
Blue LEDs represent the long-missing puzzle piece that today allows for generation of white light in combination with red and green LEDs. The invention made a large number of new applications possible, with the most important being the efficient production of white light. A modern white LED lightbulb converts more than 50 percent of the electricity it uses into light, compared to only 4 percent for incandescent bulbs. LEDs can last up to 100,000 hours – ten times longer than fluorescent lights and 100 times longer than incandescent lights. Power-efficient lighting goes a long way in terms of economic and environmental impact, and the same benefits extend to any electronic device with a LED display such as smartphones, TVs, and computers.
Applications of light: Invention of the laser
The first laser was built in 1960 by Theodore Maiman at Hughes Research Laboratories based on theoretical work by Nobel Laureates Charles H. Townes and Arthur Leonard Schawlow [6]. Less than a decade earlier, Townes suggested that stimulated emission at microwave frequencies could oscillate in a resonant cavity and produce coherent output. In 1954, his laboratory demonstrated the first maser, an acronym that stands for "microwave amplification by stimulated emission of radiation.” Serge Haroche described this accomplishment in more detail during his Lindau lecture in 2015.
(00:02:39 - 00:03:46)
Almost immediately, physicists wanted to extend the maser principle to higher frequencies. In particular, the interest was focused on amplifying stimulated emission to create coherent light with an optical oscillator. In 1958, Townes teamed up with his former colleague Schawlow to publish a detailed proposal in the journal Physical Review for what they called an “optical maser.” [7] The term laser — an acronym for “light amplification by stimulated emission of radiation” — was coined by physicist Gordon Gould, who independently came up with the design for an optical resonator.
Several groups began to work on building the first laser, but questions remained about how to excite a population inversion and what to use as an active medium. Maiman had previously made a compact microwave maser using ruby crystals and decided to work with the material again for his laser pursuits. In 1960, he tested his ruby laser design, which consisted of a ruby rod inside the coil of a photographic flash lamp enclosed in a reflective cylinder. It worked, and one of the most groundbreaking inventions of the modern age was born.
From there, continuous developments in laser technology led to more and better devices. Ali Javan and his colleagues at Bell Laboratories invented the first continuous-wave laser and the first gas laser at the end of 1960 [6]. Two years later, Robert N. Hall created the first semiconductor laser at the General Electric R&D Laboratory. At the same time, the number of laser applications grew exponentially in such wide-ranging industries such as manufacturing, telecommunications, medicine, and basic scientific research.
The earliest medical applications for lasers were in ophthalmology and dermatology. Only a year after its inventions, researchers demonstrated how the ruby laser could remove dark birthmarks and melanomas from the skin. Today, lasers remove tumors, tattoos, hair, and birthmarks in regular dermatology practice. Ophthalmologists originally used argon lasers to treat detached retinas, and later employed excimer lasers to reshape corneas and correct eyesight in LASIK procedure. A very focused laser can also cut and cauterize tissue during surgery.
In manufacturing, lasers are widely used for cutting, drilling, welding, cladding, lithography, engraving, etc. They have several advantages over traditional mechanical approaches, such as higher quality of work, high processing speed, and a longer lifetime of tools.
In the same 2015 lecture, Haroche expanded on the versatile abilities of lasers.
(00:05:20 - 00:07:16)
Laser research continues to dominate the domain of Nobel Prize achievements to this day. The 2018 Nobel Prize in Physics went to Arthur Ashkin, Gérard Mourou, and Donna Strickland for their groundbreaking inventions in the field of laser physics. Ashkin invented optical tweezers that can grab tiny objects such as particles, molecules, and even atoms with a laser beam. By using a strong lens to focus the laser light, the objects would be drawn to the point with the greatest light intensity due to radiation pressure. Optical tweezers have become the standard method for studying many biological processes – for instance, individual proteins, molecular motors, DNA, and the insides of cells.
Strickland and Mourou discovered a new technique for creating extremely short and intense laser pulses that revolutionized laser physics. Chirped pulse amplification (CPA) stretched a short laser pulse in time, amplified it, and squeezed it together again. Their invention is used in many applications, including the rapid illumination of interactions between molecules or atoms, drilling tiny holes for more efficient data storage, and manufacturing surgical stents.
Probing cells with light
In the 1970s, an early application for lasers was spectroscopy. Tunable dye lasers for spectroscopy had significant advantages over conventional sources, such as a narrow linewidth and concentrated high power in a narrow band. In 1989, Nobel Laureate William E. Moerner and German physicist Lothar Kador were the first to observe light being absorbed by a single molecule [8]. The method they invented, single-molecule spectroscopy, enables the study of what individual molecules are doing instead of millions.
A few years later, Moerner began working with variants of green fluorescent protein (GFP), a naturally occurring protein made by a jellyfish. When GFP is linked to other proteins, it can act as a visible marker, highlighting those proteins inside living cells. The 2008 Nobel Prize in Chemistry was awarded to Osamu Shimomura, Martin Chalfie, and Roger Y. Tsien for their discovery and development of GFP, which has become one of the most important tools in contemporary biology and medicine [9].
In his 2015 Lindau lecture, Moerner gave the audience an entertaining demonstration of fluorescence using a highlighter marker and a laser pointer.
(00:14:39 - 00:16:30)
Moerner built upon the initial work by discovering that the fluorescence of one variant of GFP could be turned on and off at will with two different wavelengths of light. He dispersed the excitable proteins in a gel so they were sparsely scattered, and a regular optical microscope could discern individual molecules by their glow [10]. Moerner explained details of the discovery and its relevance in his 2015 talk at Lindau.
(00:19:53 - 00:21:56)
However, the resolution of optical microscopy remained restricted by Abbe's diffraction limit — or so most researchers thought [11]. In 1873, German physicist Ernest Abbe found that the smallest distance that could be resolved under an optical microscope was roughly half the wavelength of visible light, or 0.2 micrometers. This meant that defined features of cells and microorganisms would be impossible to observe with an optical microscope and instead appear blurry.
So why not simply switch to electron microscopy, which can achieve a much higher resolution? First of all, other methods including electron microscopy involve preparation methods which kill cells and microorganisms. Optical microscopy is the only method that allows researchers to peek inside a living cell. Second, as Stefan Hell discusses in his 2016 Lindau lecture, fluorescent labeling of proteins of interest is a fairly easy and incredibly useful technique for the life sciences with optical microscopy.
(00:00:45 - 00:02:33)
Taking optical microscopy beyond the Abbe limit
Hell received the 2014 Nobel Prize in Chemistry for his work in overcoming the Abbe limit through a modified form of fluorescence microscopy, called stimulated emission depletion (STED) microscopy [10]. He had been working on the problem since 1990 and had a breakthrough after reading about stimulated emission in a book about quantum optics. In STED, one light pulse excites fluorescent molecules, while another light pulse quenches fluorescence from all molecules except those in a nanometer-sized volume in the middle. By sweeping along the sample, a full image is built up, piece by piece. The resolution of the final image will depend on the size of the volume in the middle allowed to fluoresce.
A decade later, Hell and his colleagues published a landmark paper where they announced that that Abbe's diffraction limit had been fundamentally broken [12]. They built a working STED microscope and imaged yeast cells and E. coli bacteria with a resolution of about 100 nm. In the same 2016 Lindau talk, Hell compares the before-and-after images of cellular structures with traditional optical microscopy versus STED.
(00:18:30 - 00:19:59)
Hell shared his Nobel Prize with Moerner and Eric Betzig, an American physicist who discovered a different approach to bypass the diffraction limit in optical microscopy. He initially studied near-field microscopy, which uses light emitted from an extremely thin tip placed only a few nanometers from the sample [13]. While it can obtain images of higher resolution than traditional optical microscopy, this method has a very short range and cannot visualize structures below the cell surface.
In 1995, Betzig thought of another method whereby the Abbe limit could be surpassed. If different molecules fluoresced with different colors, and all molecules of one type were dispersed and never closer to each other than 0.2 micrometers, then a microscope could register one image per color. The images put together would then result in a single, super-resolution image. Betzig spoke about his early theoretical ideas on this method — and why he abandoned them — in a 2015 Lindau lecture.
(00:06:32 - 00:08:02)
A few years later, he heard about fluorescent proteins that could be turned on and off at will and eventually realized that this was the missing link to make super-resolution microscopy a reality. Betzig and his colleagues demonstrated the technique, which they called photoactivated localization microscopy (PALM), on lysosomes and mitochondria in 2006 [14]. Since the birth of STED and PALM, super-resolution techniques have continued to improve and distinguish smaller cellular structures.
In conclusion, humanity's understanding of light and optics has grown significantly over the last 2,500 odd years — and our knowledge continues to expand at a rapid pace. Studies of the basic nature of light have led to technological advances that no one could have predicted. Medicine has taken leaps forward because of Nobel Prize winning discoveries in fluorescence and microscopy. And what will the future hold for the science of light and optics? Only time can tell, but it will undoubtedly involve more groundbreaking research by scientists in search of answers to the fundamental questions of life.
Footnotes:
[1] Stark, G. (2018). Light. In Encyclopaedia Britannica Online. Retrieved from http://www.britannica.com
[2] Vohnsen, B. (2004). A Short History of Optics. Physica Scripta. Vol. T109, 75–79
[3] Narayanan, A. (1999). Quantum Computing For Beginners. Proceedings of the 1999 Congress on Evolutionary Computation-CEC99 (Cat. No. 99TH8406)
[4] Zheludev, N. (2007). The life and times of the LED — a 100-year history. Nature Photonics. Vol. 1, 189–192
[5] https://www.nobelprize.org/prizes/physics/2014/press-release/
[6] Hecht, J. (2010). Short history of laser development. Optical Engineering. Vol. 49, F100-F122.
[7] Schawlow, A. L. and Townes, C. H. (1958) Infrared and Optical Masers. Physical Review. Vol. 112, 1940-1949
[8] Moerner, W. E. and Kador, L. (1989) Optical detection and spectroscopy of single molecules in a solid. Physical Review Letters. Vol. 62, 2535–2538
[9] https://www.nobelprize.org/prizes/chemistry/2008/summary/
[10] https://assets.nobelprize.org/uploads/2018/06/popular-chemistryprize2014.pdf
[11] Stelzer, E. H. K. (2002) Light microscopy: Beyond the diffraction limit? Nature. Vol. 417, 806-807
[12] Klar, T. A., Jakobs, S., Dyba, M., Egner, A. and Hell, S. W. (2000) Fluorescence microscopy with diffraction resolution barrier broken by stimulated emission. PNAS. Vol. 97, 8206-8210
[13] Betzig, E. and Trautman, J. K. (1992) Near-Field Optics: Microscopy, Spectroscopy, and Surface Modification Beyond the Diffraction Limit. Science. Vol. 257, 189-195
[14] Betzig, E. et al. (2006) Imaging Intracellular Fluorescent Proteins at Nanometer Resolution. Science. Vol. 313, 1642-1645