Prof. Dr. Carlo Rubbia > Research Profile
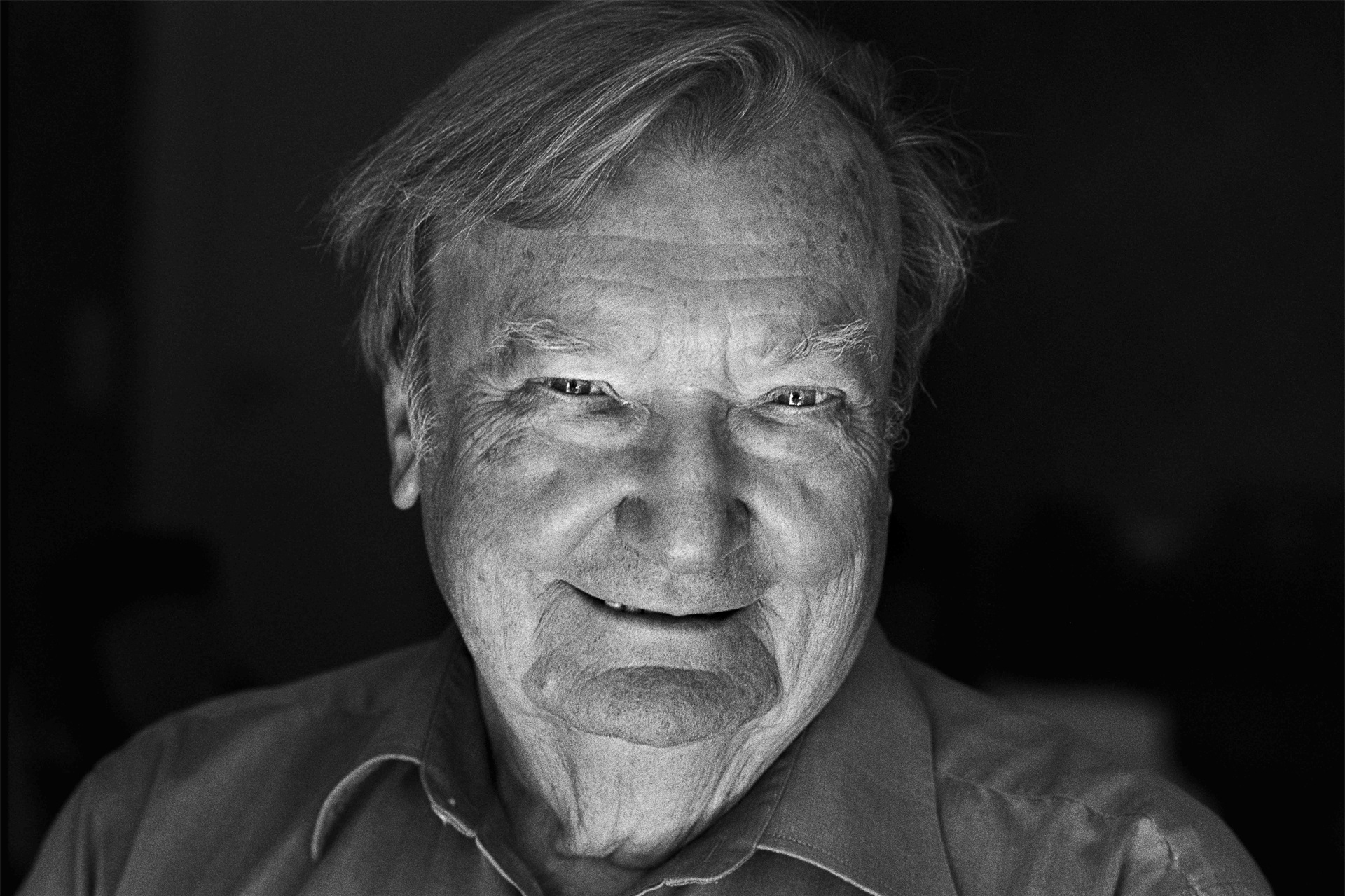
by Roberto Lalli
Carlo Rubbia
Nobel Prize in Physics 1984 together with Simon van der Meer
"for their decisive contributions to the large project, which led to the discovery of the field particles W and Z, communicators of weak interaction".
A Passion for Applied Physics and Technology
Carlo Rubbia was born in Gorizia, Italy, on 31 March 1934, son to an electrical engineer and an elementary school teacher. Since an early age, Rubbia showed a strong inclination towards natural sciences and was especially fascinated by electronics. The applied aspect of physics was so relevant for him that he was undecided whether he would become a physicist or an engineer. Rubbia chose physics, and, in early 1951, he applied to the physics degree course of the prestigious Scuola Normale Superiore of Pisa (SMS)—a very selective special-statute Italian public university that accepted only ten physics students per year out of more than five hundreds applicants. Chance had an important role in the continuation of Rubbia’s career in physics. His application was initially rejected for he arrived only eleventh in the selection process. After having started the engineering course at the Polytechnic University of Milan, however, he was re-admitted into the SMS because one of the winning students had resigned. From that moment onward, Rubbia did not abandon the world of physics again.
In 1958, Rubbia obtained a M.Sc. degree in physics with a thesis on cosmic-ray physics. Rubbia’s thesis advisor was the well-known Italian experimental physicist Marcello Conversi. During the war, Conversi had collaborated in an important experiment, which showed that the cosmic-ray mesotron could not be the particle predicted by H. Yukawa as the carrier of the force that holds the nucleus together.
Upon graduation, Rubbia spent one and a half years and half at Columbia University as Fulbright Fellow to acquire experience with the novel techniques employed in American particle accelerators. He worked with W. F. Baker on the decay and nuclear capture of muons at the synchrocyclotron of the Nevis Laboratories—an accelerator operated by the University of Chicago physics department. In these experiments, Rubbia and Baker observed a spatial asymmetry in the angular distribution of the neutrons emitted after the capture of polarized negative muons, which confirmed the recent discovery that parity was violated in weak interactions. Rubbia’s experience at Nevis Labs marked his entry in the field of weak interactions, which remained the central focus of his research endeavours in the following years.
Building his Leadership at CERN
Around 1960, Rubbia decided to come back to Europe to work in the laboratories of the European Organization of Nuclear Research (CERN). The CERN was established in 1954 by twelve member states of Western Europe (including, among others, U.K., France, Italy, and West Germany) with the aim to operate large particle accelerators built around the Swiss-French border. The Austrian-born theoretical physicist V. Weisskopf—Director General of CERN from 1961 to 1965—soon promoted Rubbia to group leader, making him one of the youngest group leaders in the growing community of particle physics. At CERN, Rubbia ideated and led several experiments with the 600 MeV Syncrocyclotron devoted to the understanding of the properties of the weak interaction. Although still in his twenties, Rubbia demonstrated strong leadership skills as well as volcanic energy. Among the main results obtained by Rubbia and his co-workers in the early 1960s, one finds the discovery of the beta decay of the positive pion and the first observation of muon capture by pure hydrogen. Rubbia also had the opportunity to employ the newly built 28 GeV Proton Synchrotron (PS), producing some interesting results about the non-conservation of parity in the beta decay of lambda hyperons.
After the unexpected discovery of the slight violation of CP symmetry in weak interactions by Fitch and Cronin in 1964, Rubbia began working on this topic in collaboration with the American physicist J. Steinberger, who had contributed to the discovery that there existed two different kinds of neutrinos (the electron neutrinos and the muon neutrinos) two years earlier. Between 1965 and 1967, Rubbia, Steinberger and their collaborators made a series of experiments on the decay properties of the neutral mesons at the PS, which confirmed the non-conservation of CP symmetry. Rubbia continued to pursue similar kinds of experiments in collaboration with other physicists until 1970.
Between United States and Europe
In 1970, Carlo Rubbia was offered a full professorship at Harvard University, which he accepted. This professional change allowed Rubbia to pursue experimental projects both in the United States and in Europe. The international competition between research groups and national governments to be at the forefront in particle physics research was leading to the construction of more and more powerful accelerators. Since the late 1960s, a new facility was under construction in Illinois, about 50 km west of Chicago. The National Accelerator Laboratory (later christened Fermi National Accelerator Laboratory, or Fermilab for short) was planned to host a proton synchrotron able to accelerate protons up to 500 GeV—about 10 times the energy reached by the major high-energy proton accelerators of the period. Experimental particle physicists knew that the new accelerator would be a great advancement in the pursuit of answering fundamental questions about the behaviour of elementary particles.
The Western European countries did not stay behind, though. In 1971, the first hadron collider ever built—the Intersecting Storage Ring (ISR)—began operations at CERN. The ISR (in conjunction with the 28 GeV Proton Synchrotron) could accelerate protons up to 31 GeV for a total centre-of-mass energy of 62 GeV. In the early 1970s, Rubbia participated in a series of experiments on the determination of the proton-proton elastic cross section at the high centre-of-mass energies reached at the ISR. These experiments became fundamental for at least two correlated reasons. The first was that the experimental teams began employing a method for the phase-space compression of proton beams developed in 1968 by the Dutch physicist Simon van der Meer (called stochastic cooling). The second was that, also thanks to this method, the experiments obtained a very high luminosity, i.e., a large ratio of detected events in a certain time to the interaction cross section.
In the meantime, Rubbia was pursuing other projects concerning weak interactions in the United States. After the discovery of parity violation in 1957, two groups of theoretical physicists developed, independently, a theory that described the weak interactions as a combination of vector and axial vector currents (the V-A theory). The theory predicted that weak interactions were mediated by bosons in the same way as photons mediate electromagnetic interactions in Quantum Electrodynamics. The intermediate vector bosons of weak interactions were initially called W (for weak).
In 1970, Rubbia, along with David Cline (University of Wisconsin) and Alfred Mann (University of Pennsylvania), proposed an experiment with high-energy neutrinos in order to provide evidence for the existence of the hypothetical W bosons by analysing their hadronic decay. The three physicists proposed the high-energy neutrino experiment to be performed at the proton synchrotron that was under construction at Fermilab. One year later, momentous advancements in the theory of weak interactions made the proposed energetic neutrino experiment crucial for a different reason, which was not a priority when the experiment was proposed. In 1967 and 1968, Weinberg and, independently, Salam put forward a theory for the unification of electromagnetic and weak interactions that extended a model published by S. Glashow in 1961. The theory predicted that weak interactions were mediated by three very heavy bosons: the charged W+ and W- and the neutral Z. With respect to other theories of weak interactions, the Glashow-Weinberg-Salam SU(2)xU(1) gauge theory, therefore, predicted the existence of weak neutral currents carried by the heavy boson Z besides the charged currents mediated by the W’s. However, neutral currents had never been detected. It was expected that, if Z existed, the best way to identify neutral currents was to look at the decays of a K-meson into a pion of the same charge and a lepton-antilepton pair. Such a decay does not conserve strangeness, and since strangeness is conserved in electromagnetic and strong interactions, the detection of these processes would have demonstrated the existence of weak neutral currents (i.e., charge is conserved in the hadronic and in the leptonic current separately). None of these hypothetical processes was ever observed and, in the 1960s, the consensus that neutral currents did not exist in nature was very widespread. In 1970, Glashow, J. Iliopoulos and L. Maiani elaborated a mechanism (GIM mechanism) that entailed the suppression of flavour-changing neutral currents. This mechanism was related to the Glashow-Weinberg-Salam model after ‘t Hooft demonstrated that a large set of gauge theories (including the Weinberg-Salam theory) was renormalizable. These theoretical advancements shortly made the SU(2)xU(1) electroweak theory plus the GIM mechanism one of the major candidates for the description of weak interactions.
Some theorists pushed experimental physicists to dedicate more efforts to the search for neutral currents with the high-intensity high-energy beams of neutrinos produced in the powerful proton synchrotrons. The almost massless (at the time it was broadly believed that neutrinos’ mass was null) and uncharged neutrinos reacted only through weak interactions. Therefore, they constituted a perfect means to detect the neutral currents, if they existed.
After the 1962 discovery that there were two different kinds of neutrinos, it was understood that the neutrinos produced in proton accelerators were muon neutrinos. In the early 1970s, several high-energy neutrino experiments had led to the observation of charged weak interactions in which muon neutrinos turned into muons after interacting with electrons or hadrons. In order to observe neutral currents, it was thought to look for reactions in which the muon neutrino did not change its identity after a weak interaction. The experiment was particularly challenging because neutrinos cannot be directly detected. The behaviour of neutrinos could be inferred only by investigating the trajectories of the other interacting particles (either electrons or hadrons).
Once the importance of neutral currents had become clear, Rubbia and his collaborators included the search for these events in the goals of the high-energy neutrino experiment under development at Fermilab. At the beginning of 1973, a 13-physicist team from four different institutions (Harvard, Pennsylvania, Wisconsin, Fermilab, HPWF collaboration for short) began making observations, but the analysis of the data did not allow the investigators to reach a definitive conclusion concerning the existence of neutral currents in the next few months. Meanwhile, an experiment that was being performed at CERN with a 12-m3 bubble chamber called Gargamelle (after Gargantua’s mother) could announce a final result by mid-1973. After much internal discussion, the CERN collaboration concluded that they had persuasive evidence for weak neutral currents in their bubble-chamber images. The HPWF collaboration initially did not corroborate the Gargamelle finding, but in 1974, after much hesitation and a further set of observations, the HPWF collaboration confirmed that their observations also showed evidence for the existence of neutral currents. This independent confirmation provided strong support to the discovery of one of the most striking predictions of the Glashow-Weinberg-Salam model of electroweak interactions.
In 1974, Rubbia also speculated that the HPWF experiment had showed evidence for a couple of dimuonic events—reactions in which a pair of muons is produced—that could be interpreted as evidence of a new hadronic quantum number. However, the number of events was too low to be persuasive. In November 1974, two different experiments performed respectively at the Brookhaven National Laboratory (BNL) and Stanford Linear Accelerator Laboratory (SLAC) discovered a particle—later known as J/Psi—composed of a new quark (the charmed quark) and its antiparticle. The November revolution, as the discovery of the J/Psi particle was later celebrated, was considered one of the most important events in early 1970s particle physics research, providing a strong support to the validity of the Weinberg-Salam theory of electroweak interactions extended with the GIM mechanism. After the discovery was announced, it was deduced that Rubbia and his collaborators had observed the same particle in their neutrino experiments. The discovery of the J/Psi was considered so relevant that the leaders of the two experimental teams involved in the discovery—S. Ting and B. Richter—were awarded the Physics Nobel Prize in 1976, only two years after the announcement of the existence of the J/Psi particle.
The Proton-Antiproton Collider
After the discovery of neutral currents and of the charm quark, one of the more urgent experimental endeavours became to provide direct evidence for the existence of the three intermediate vector bosons—the W and Z particles. The detection of these massive bosons was, however, extremely complex. Calculation in the framework of the Weinberg-Salam theory predicted that the mediating bosons were highly massive (between 50 and 100 GeV). While they naturally existed in the virtual state as mediators of the weak force, in order to produce them in a real state it an extremely high amount of energy was necessary —much higher than the upper limit reached by the accelerators available in the mid-1970s. Since 1972, the Fermilab proton synchrotron accelerated protons up to 500 GeV, but the energy available for the production of new particles did not reach 50 GeV. Because of the conservation of the total linear momentum, most of the kinetic energy of the accelerated particles remains in the form of linear momentum of the particles emitted after the collision.
To reach a greater energy it was more efficient to employ colliders that accelerated particles in counter-rotating rings. After the frontal collision the total momentum is very low because the interacting particles had approximately the same velocity in opposite directions. Most of the energy of the two colliding particles was, then, available for the creation of new particles. In the mid-1970s, however, no collider had the centre-of-mass energy sufficient to the production of the W and Z particles.
To solve this issue, Rubbia elaborated an idea initially proposed by Peter McIntyre to convert high-energy proton synchrotrons in proton-antiproton colliders. A device of this kind would have permitted reaching very high energies and produced collisions that were in principle able to generate the intermediate vector bosons. When Rubbia conceived the project, the SU(2)xU(1) gauge theory of electroweak interactions and the SU(3) gauge theory of strong interactions of quarks and gluons, called quantum chromodynamics (QCD), were gaining momentum. In the framework of these theories, the collision between one proton and one antiproton was a very complex interaction of many different particles. Among this range of possibilities, there were some collisions able to produce intermediate vector bosons in case they were energetic enough. Theoretically, the neutral Z boson could be produced by a collision between a quark and an antiquark of the same flavour, while the charged W bosons by a collision between a quark and an antiquark of two different flavours. Although quarks (antiquarks) carry only a fraction of the total energy of the accelerated protons (antiprotons), it was calculated that centre-of-mass energies around 270 GeV would have been sufficient to make the intermediate vector bosons detectable. There were two accelerators that, if converted to colliders, could have reached such energy: the 500 GeV Fermilab proton synchrotron and the 400 GeV Super Positron Synchrotron (SPS) at CERN, which began operation in 1976.
That same year, Rubbia, along with Cline and McIntyre, put forward a proposal for the conversion of the existing synchrotrons to proton-antiproton colliders. In principle, the idea was rather simple. It did not require a radical modification of the facilities because the mechanism that accelerated protons in one direction also accelerated antiprotons in the opposite direction. The major difficulty of the entire scheme was to produce a beam of antiprotons compact enough to produce the required amount of collisions.
High-energy antiprotons could be generated by the collisions of proton beams on a fixed target. The experimental project required, however, that these antiprotons were produced in an industrial number (10^11 per morning) and compressed in space and phase (cooled) in such a way that they could remain compact for the entire day after being injected into the SPS. In 1966, the Russian physicist Gersch Budker had developed a method for cooling protons by employing electrons to reduce their momentum variation. The method was tested successfully only in 1976 and soon the Fermilab began testing whether it was possible to utilize the electronic cooling to transform the proton synchrotron into a proton-antiproton collider in the near future. However, the Fermilab management had already decided to embark on the ambitious project to increase the maximum energy of the facility from 500 GeV to 1 TeV, employing giant superconducting magnets. The directorship of the Fermilab decided that priority should be given to this project, before any further transformation was done.
At CERN, the 400 GeV SPS had just begun operation when the proposal of the conversion was submitted. The directors of CERN, Sir John Adams and Leon van Hove, were convinced, not least by Rubbia’s strong and passionate commitment, to support the project although there were several unknowns. One of the major reasons underlying the risky attitude of CERN directors was that CERN had not made until then any fundamental discovery in the field of particle physics. In spite of the many efforts undertaken, the major discoveries had been made in American laboratories, apart from the discovery of neutral currents. The latter discovery, however, had not been awarded with a Nobel Prize, which was considered the major recognition for scientific achievements. CERN directors hoped that the discovery of the intermediate vector bosons would be the major discovery they had been waiting for since the CERN was established twenty years earlier.
Before undertaking the transformation of the synchrotron into a collider, it was decided to test der Meer’s stochastic cooling method in order to see if it were possible to create antiproton beams intense enough to be efficient for the proposed experiment. The Initial Cooling Experiment (ICE project), as the test was called, began in December 1977. After the project led to the understanding that the stochastic cooling could be indeed practicable, the CERN management decided to start off the collider project in June 1978.
The UA1-UA2 Competition
Rubbia projected a giant machine to detect the particles emitted from the head-on collisions of protons and antiprotons. Rubbia’s detector was very ambitious: it consisted of a innovative drift chamber for the precise measurement of the momentum of a charged particle (called central detector, or CD for short), a set of electromagnetic and hadronic calorimeters for the detection of the energies of both leptons and hadrons, and long drift tubes able to show the trajectories of muons.
The project was accepted and started under the name Underground Area 1 (UA1). Rubbia became the spokesperson of the UA1 collaboration, which comprised about 150 physicists in different groups, coming from Austria, France, Germany, Italy, the United Kingdom, and the United States. The various groups of the UA1 collaboration took the responsibility to build different sections of the detector. The group at CERN built the CD, which was the main, and most critical, part of the detector. Rubbia headed the efforts of the CERN group and was actively in charge of developing the complex electronics of the CD.
The UA1 experiment was not the only planned experiment for the detection of the W and Z bosons at CERN. The CERN management wanted a different detector to be built in a different intersecting point of the 22km-long SPS collider. Two different experiments would have allowed a rapid confirmation of the findings made by the other detector or, at worst, provided some evidence in case one of the two detectors did not work properly. A rather traditional experiment was chosen (at least with respect to the ambitious innovations of the UA1 project), called UA2. Led by Pierre Darriulat, the UA2 experiment became a strong competitor to the UA1 experiment. After the two projects were approved a race between them began in order to produce as quickly as possible persuasive results and, consequently, to claim priority for the discovery of the sought-after particles.
The Discovery of the W and the Z
In July 1981, the SPS was used for the first time to accelerate counter-rotating beams of protons and antiprotons. At this early stage, the proton beam contained many more particles than the antiproton beam. The major difficulty was to distinguish a real proton-antiproton interaction from the background constituted by proton-gas collisions. A way to do that was to focus on the time delay between the detected events in different directions. A proton-antiproton collision was expected to produce events that would be detected at the same time by two scintillation counters positioned at the front and the back of the detector. Employing this criterion, the first proton-antiproton collision was detected at CERN after two days of operation with counter-rotating beams. The intensity of the antiproton beam was however too low (by a factor of 100) to allow the detection of intermediate vector bosons. Moreover, the UA1 collaboration had not completed its own detector. Both the UA1 and UA2 collaborations as well as the CERN scientists working at improving the intensity of antiproton beams worked frenetically to solve the various technical issues.
To complete the necessary improvements took about one year, also because of some incidents. In October 1982, a new run began. Both the UA1 and the UA2 had been completed and successfully tested. The SPS accelerated counter-rotating beams of protons and anti-protons to 270 GeV for a total centre-of-mass energy of 540 GeV. The intensity of the antiproton beams was great enough to allow in principle the detection of a few decays of W and Z particles.
The investigators decided to begin looking for evidence for the W bosons, which were produced more abundantly than the Z boson. Although charged, the lifetime of the W particles is so short that they cannot be directly detected. Among the various decays of the W boson the investigators were hoping to detect a characteristic decay—often called signature—that could signal the presence of a W. They were looking for a small number of golden events that could be explained only as decays of W bosons. The preferred one was the emission of one electron (positron) and the related electron antineutrino (neutrino) with elevated transverse energies. Neutrinos, however, could not be directly detected. Hence, the presence of neutrinos with high transverse energy should be deduced by the transverse energy missed calculating the energy of all the decay products of one proton-antiproton collision. That was the reason underlying the design of the detector, which was uniformly sensitive over the whole solid angle (with tiny gaps that made some evidence inconclusive) to all the charged and neutral interacting debris produced by the proton-antiproton collision.
By the end of the run, in December 1982, the UA1 collaboration had found 6 good W boson candidates without any jet activity and with large missing momenta. After the UA2 confirmed that they also had found a slightly smaller amount of candidates, Rubbia felt free to make the formal announcement and quickly published the paper announcing the discovery in February 1982. The UA2 officially confirmed the finding of the W particle with their detector one month later. Both the experiments found a mass for the W boson around 85 GeV in full agreement with the prediction of the Weinberg-Salam theory.
After the winter shutdown, operation began again in April 1983. Now, the intensity and stability of antiproton bunches had reached optimal levels. With such a source the discovery of signatures of the W boson became almost a routine. As a consequence, the UA1 and UA2 teams directed their efforts to the discovery of the Z particle. The neutral Z boson could be discovered by looking at the simultaneous emission of high transverse energy electron-positron or muon-antimuon pairs. On 4 May 1983, the first Z candidate was found in the analysis of a collision that had emitted an electron-positron pair with high transverse energy. The mass of the first Z candidate was calculated to be about 100 GeV in perfect agreement with what was expected on the basis of the Weinberg-Salam theory. Although the momentum of one of the two tracks was less than expected, Rubbia and his collaborators considered this event as the first good evidence of the existence of the Z boson. The recently appointed CERN Director General, Herwig Schopper, immediately announced the discovery at an important conference he was attending. A few days later, a second candidate appeared in a high-energy muonic event. This time, the evidence was much better and a paper was rapidly published announcing the discovery of the neutral intermediate vector boson.
The Glashow-Weinberg-Salam model of electroweak interaction received in this way a strong support and the discovery was soon celebrated around the world. One year later, Rubbia and S. van der Meer were awarded the Physics Nobel Prize “for their decisive contributions to the large project, which led to the discovery of the field particles W and Z, communicators of weak interaction.” As the 1984 Nobel Prize press release rightly underlined, the project was the largest for involved number of people ever awarded with a Nobel Prize. The Nobel committee decided to reward two persons out of this large cooperative effort: “Carlo Rubbia, who had and developed the idea, and Simon Van der Meer, whose invention made it feasible.”
The social responsibility of a physicist
Some of the detected events led the UA1 physicsts to think that they were close to another important discovery, which could undermine the validity of the Standard Model of particle physics and provide evidence for the validity of supersymmetric theories. One year of work in defining the background made it clear, however, that all the events detected at the SPS collider were in accord with the Weinberg-Salam theory of electroweak interaction and QCD. In the following years, the SPS collider was at the forefront in the empirical study of strong interactions. The machine lost its leading role only around 1986, when the newly built Fermilab Tetravon produced the first proton-antiproton collision at 1.8 TeV.
Rubbia continued to hold a leadership position in various CERN projects and in international collaborations. He was among the promoters of an experiment performed at the Laboratori Nazionali del Gran Sasso (LNGS) in Italy to test the hypothetical proton decay. According to the Standard Model, the proton is indefinitely stable because it is the lightest of baryons and the conservation of the baryonic number does not allow the proton to decay into other particles. Some unified theories predict instead a finite (albeit extremely large) time of decay. The LNGS experiment was designed to test this hypothesis, but it did not provide any evidence of proton decay.
In 1989, Rubbia became Director General of CERN—a position he held till 1993. During these years, he saw the early experiments performed at the Large Electron-Positron Collider (LEP). In the meantime, he continued to develop the project of the Large Hadron Collider (LHC), which was to become the largest and most powerful particle collider in the World. In 1993, at the end of Rubbia’s directorship, the project got accepted and construction began the following year through a collaboration of about 10000 scientists from over 100 countries.
The construction of the LHC took more than a decade, and the facility began operating in 2008. After the resolution of various technical problems, the accelerator allowed the discovery of the long-sought Higgs boson in 2012. In 2013, the theoretical physicists François Englert and Peter W. Higgs, who had predicted its existence around 1964, were jointly awarded the for this discovery. This reward led to some questions whether an institution (namely, the CERN) could also been awarded with a Nobel Prize, since the momentous experimental endeavour itself, so the arguments go, had not received the recognition it deserved. The LHC had been strongly promoted by Rubbia, and his Nobel Prize had probably helped him to gain the necessary political and economical support to build the giant accelerator.
In the last years, Rubbia has been strongly concerned with topics not strictly related to particle physics and its developments. He has been involved in numerous projects to face the perils of climate change and the shortage of energy, participating in several advisory committees and proposing innovative solutions. Rubbia believes it to be the social responsibility of scientists to be involved in trying to solve these issues, which arguably constitute the greatest challenges facing humanity. For this commitment as well as for his scientific achievements, Rubbia has been appointed Senator for life of the Italian Republic in 2013.
Bibliography
Fitch V. L., & Rosner L. (1995) Elementary Particle Physics in the Second Half of the Twentieth Century. In Brown, L., Pippard, B., & Pais, A. (Eds.). Twentieth Century Physics (Vol. 2). AIP, New York, pp. 635-794.
Galison, P. (1987) How Experiments End. University of Chicago Press, Chicago.
Hoddeson, L. Brown, L. M., Riordan, M. & Dresden, M. (1997) The Rise of the Standard Model: Particle Physics in the 1960s and the 1970s. Cambridge University Press, Cambridge.
Krige, J. (ed.) (1996) History of CERN, Vol. 3. Elsevier, Amsterdam.
Press Release: The 1984 Nobel Prize in Physics". Nobelprize.org. Nobel Media AB 2014. Accessed 1 October 2014. <http://www.nobelprize.org/nobel_prizes/physics/laureates/1984/press.html>
Rubbia, C. (1984) Carlo Rubbia - Biographical. Nobelprize.org. Nobel Media AB 2014. Accessed 30 August 2014. <http://www.nobelprize.org/nobel_prizes/physics/laureates/1984/rubbia-bio.html>
Rubbia, C. (1985). Experimental Observation of the Intermediate Vector Bosons W+, W-and Z0. Reviews of Modern Physics 57, pp. 699-722
Rubbia, C. (2008) Carlo Rubbia – Interview, July 2008. Nobelprize.org. Nobel Media AB 2014. Accessed 1 October 2014. <http://www.nobelprize.org/nobel_prizes/physics/laureates/1984/rubbia-interview.html>
Sessler A. and Wilson E. (2007) Engines of Discovery: A Century of Particle Accelerators. World Scientific, Singapore.
Taubes, G. (1986) Nobel Dreams: Power, Deceit, and the Ultimate Experiment. Random House, New York.
van der Meer, S. (1985) Stochastic cooling and the accumulation of antiprotons. Reviews of Modern Physics 57, pp. 689-700
Watkins, P. (1986) Story of the W and Z. Cambridge University Press, Cambridge.